DOI: https://doi.org/10.56669/CDMA8114
ABSTRACT
Modern cropping systems rely heavily on chemical or synthetic fertilizers to increase crop yields, but their long-term and continuous application can have detrimental effects on the environment, soil biota, and fertility. These issues emphasize the need for highly efficient, cost-effective fertilizers that can increase yields while minimizing environmental damage. As a non-toxic and eco-friendly alternative, bio- and nano-fertilizers are preferred over synthetic (chemical) fertilizers to ensure biosafety in agriculture. In recent years, the use of a combination of nanoparticles and biofertilizer, referred to as nano-biofertilizer (biofertilizer coated with nanomaterial) has been promoted to combine the synergism of individuals for sustainable crop production. While the use of nano-biofertilizer is currently in its infancy phase in agriculture, it has the potential to transform traditional agriculture into smart one. In this review, we provide a comprehensive overview of nano-biofertilizer formulation, characterization, and application, and elucidate the underlying mechanisms by which they promote plant growth and how they help plants cope with biotic and abiotic stresses caused by climate change. We summarize the latest advances of nano-biofertilizer applications in precision agriculture. The limitations and future strategies for formulating effective nano-biofertilizer are discussed.
Keywords: nanoparticles, biofertilizers, plant growth promotion, phytohormones, and biocontrol of phytopathogens
INTRODUCTION
Since the green revolution, agrochemicals (chemical fertilizers and pesticides) have become an indispensable part of intensive cropping systems. Although agrochemicals boost crop yields, their persistent and prolonged use has been shown to have detrimental effects on future agricultural prospects, causing environmental pollution, including greenhouse gas emissions and global warming, soil acidification and degradation, eutrophication of water, loss of biodiversity, emergence of pests, nutrient loss and its biomagnification in food chains, which can pose a health threat (Kah et al., 2019). The exploration of alternative nutrient solutions and modification of existing nutrient sources are therefore crucial for ensuring agricultural sustainability of crop production.
Over the past decade, a great deal of effort has been put into replacing chemical fertilizers with better eco-friendly and cost-effective alternatives. A promising alternative to chemical fertilizers for sustainable agriculture is using plant growth promoting rhizobacteria (PGPRs), endo- and ectomycorrhizal fungi, cyanobacteria and many other useful microscopic organisms as biofertilizers (Kumar et al., 2022). The increased availability of plant nutrients (e.g., N, P, K, and Zn), the modulation of phytohormones (e.g., indoleacetic acid and gibberellic acid), the biocontrol of phytopathogens, and the amelioration of biotic and abiotic stresses are some of the mechanisms by which plant growth and yield are improved in response to biofertilizer inoculation (Kumar et al., 2022). Biofertilizers, however, present a number of challenges, such as poor shelf-life, on-field stability, performance under fluctuating climatic conditions, and the most significant issue is high dosage requirements. In recent years, nanotechnology has opened up unprecedented possibilities for agricultural sustainability and intensive production practices through the development of nanofertilizers and nanopesticides (Kah et al., 2018). Owing to its distinct characteristics such as high surface area, high solubility, and lightness, nanoparticle (NP)-based formulation has embraced many implementation domains in agriculture, such as improved nutrient use efficiency, enhanced plant growth, and disease resistance, making it preferable compared to synthetic fertilizers and biofertilizers (Kah et al., 2018, 2019). By integrating nanotechnology and biotechnology, modern agriculture strives to make farming more efficient, cost-effective, and eco-sustainable while ensuring food security. Nano-biofertilizer (NBF), a hybrid of nanoparticle (NP) and biofertilizer that produces a more potent option for eco-sustainable agriculture, represents an advancement in agricultural innovation (Sharma et al., 2023). NBFs are made by encapsulating biofertilizers reduced to nanoscale (1-100 nm) within nanomaterial coatings. It overcomes the drawbacks of biofertilizers, reduces the dependency on chemical fertilizers, and combats major issues of crop production, food security, sustainability and eco-safety. NBFs provide a number of important advantages, including: (i) slow and steady release of nutrient to crop plants without any unintended loss; (ii) increased nutrient use efficiency; (iii) enriched beneficial microorganisms in soils; (iv) improved disease resistance of crops; (v) mass production in less time; (vi) cost effectiveness; and (vii) renewable fertilization (Sharma et al., 2023). Yet, the application of NBF is advancing from the experimental to the practical realms. Due to the limited understanding of NBF synthesis, especially the green synthesis of NBFs, their effective application methods, and the mechanisms by which NBFs mediate plant growth, they have not been widely endorsed and commercialized. This review article explores the formulation, characterization, and application of NBFs, as well as unraveling the mechanisms by which they promote plant growth and cope with climate change-induced stresses. We provide a summary of the most recent developments in the use of NBFs in precision agriculture. The disadvantages and potential approaches for developing effective NBFs are discussed.
Formulation and characterization of NBFs
Nano-biofertilizer formulation mainly involves top-down and bottom-up approaches. In top-down approach, NBFs are produced from large-sized raw components, whose size is reduced by various mechano-physical procedures, whereas bottom-up approaches develop NPs from atoms, compounds, or smaller particles through chemical and biological reactions. A wide variety of mechano-physicochemical techniques, such as mechanical grinding/milling, co-precipitation, chemical etching, sputtering, lithography, spray pyrolysis, laser pyrolysis, evaporation-condensation, sol-gel, photochemical reduction, and laser ablation techniques have been used in top-down and bottom-up approaches for the synthesis of metal NPs (Jamkhande et al., 2019). In general, NBFs are synthesized by physicochemical methods and biological methods (bottom-up), although the latter has emerged as a fast, measurable, non-toxic, energy efficient and economically viable method (Sharma et al., 2023).
In biological methods for NP synthesis, microorganisms are commonly used because they release inorganic materials either intracellularly or extracellularly, such as magnetostatic bacteria that release magnetite, diatoms that release silicious materials, and S-layer bacteria that release calcium carbonate and gypsum (Singh et al., 2016). For the intracellular synthesis of NPs, microorganisms take metal ions from the surrounding environment and convert them to elemental forms using enzyme reduction, while for the extracellular synthesis of NPs, microorganisms produce proteins and enzymes that reduce metal ions and make them stable (Bahrulolum et al., 2021). In general, biological methods offer more control and management over crystal shape, size, composition, and structural stabilization of NP while putting an end to the requirement for reactive and toxic reducing chemicals, which are hazardous to microorganisms (Bahrulolum et al., 2021).
In recent years, the green synthesis of NBFs has emerged as an efficient, eco-friendly, and economically feasible method with a number of advantages over conventionally produced NBFs (Bairwa et al., 2023). Green NBFs are synthesized using biogenic and microbial compounds such as plant (stem, root, seed, leaf, fruit, flower) extracts, fermented extracts, enzymes, organic polymers (extracellular polymeric substance) and other polysaccharides as reducing agents in bionanofactories where metal ions are reduced to create biocompatible nanocomposites. A wide range of microorganisms, such as plant beneficial-bacteria like Clostridium thermoaceticum, Desulfovibrio desulfuricans, Klebsiella aerogenes, cyanobacteria like Spirulina, Nostoc, Anabaena, Synechocystis, Phormidium, Lyngbya, Oscillatoria and green algae like Chlorella, Scenedesmus, Chlorococcum, Cosmarium, Chlamydomonas, Botryococcus have been used as potential biofactories to synthesize a variety of metal NPs containing metals like silver, gold, copper, zinc, titanium, palladium, and nickel in an economical and environmentally friendly way (Bairwa et al., 2023; Sharma et al., 2023). Recently, myconanotechnology has emerged as a promising research area in which fungi (e.g., Aspergillus sp., Fusarium oxysporum) can be used to synthesize metal NPs both intracellularly and extracellularly. Due to their greater efficacy and widespread distribution in nature, fungi have been chosen over other microorganisms for the synthesis of metal NPs (Sonawane et al., 2022).
The synthesis of NBF involves three important steps: (1) the growth of the biofertilizer culture; (2) its encapsulation with NPs; and (3) evaluation and assessment of its performance, quality, purity, and self-life (Bairwa et al., 2023). For the production of fungal NBF, MGYP broth (maltose, glucose, yeast potato) was used to culture the fungus (Alternaria brassicae) for 5 days at 27 °C and the fungal colonies were recovered by sieving. By adding AgNO3 to harvested biomass and incubating in the dark, silver-nanoparticles (AgNPs) were produced. A UV–VIS spectrophotometer (350–650 nm) was used to test and validate the produced AgNPs, which were then freeze-dried and stored for use in the future (Fatima et al., 2018). NBFs are increasingly being synthesized using cell-free supernatant from bacteria. It can also be synthesized in the form of microcapsule. For this, plant growth promoting rhizobacteria (PGPR) suspension was mixed with 1.5% sodium alginate, 3% starch, and 4% bentonite in a 2:1 ratio. After the mixture was coated with calcium chloride solution, the microcapsules were washed with sterile distilled water. Mono- and bimetallic iron and manganese NPs were successfully synthesized using the endophyte bacterium Paenibacillus polymyxa supernatant solution with auxin (IAA) (de França Bettencourt et al., 2020). Several factors, including microbial origin, reaction temperature, pH, pressure, incubation time, and metal salt concentration, control the synthesis of NBFs.
Nanoparticles used in the synthesis of NBFs
Nanoparticles commonly used for NBFs formulation are silicon (Si), iron (Fe), copper (Cu), zinc (Zn), silver (Ag), and titanium (Ti). The mechanisms through which various NPs enhance plant growth vary. The constituents of NBFs (NPs + biofertilizer), targeted plant, mode of application, and response of plants to NBFs are shown in Table 1.
Table 1. The constituents of nano-biofertilizer (NBF), targeted plant, mode of application, and response of plants to NBFs
Nanobiofertilizer
|
Targeted plant
|
Mode of application
|
Condition
|
Plant responses
|
References
|
Nano particle
|
Biological component
|
Si
|
Pseudomonas fluorescens,
P. putida
|
Melissa officinalis L.
|
Seed priming
|
Normal
|
Increased photosynthetic pigments, total soluble protein and phenolic content, leaf water content, and plant biomass
|
Hatami et al.
(2021)
|
SiO2
|
Azospirillum brasilense,
Bacillus sp., Azospirillum
lipoferum
|
Triticum aestivum
|
Soil
|
Drought
|
Increased photosynthetic pigments, osmolytes content, relative water content, membrane stability index, phenol, and flavonoid content, indole acetic acid and cytokinin content, germination percentage, germination index, and yield
|
Akhtar and Ilyas
(2022)
|
Fe (zero-valent)
|
Compost and biochar
|
Brassica juncea L.
|
Soil
|
Heavy metal
stress
|
Enhanced soil cation exchange capacity, soil pH, total nitrogen and total carbon, and plant biomass
|
Baragano et al.
(2020)
|
Fe (zero-valent)
|
Pseudomonas
fluorescens
|
Trifolium repens
|
Soil
|
Antimony stress
|
Improved plant growth and phytoremediation
potential
|
Daryabeigi Zand
et al. (2020)
|
Cu
|
Streptomyces griseus
|
Tea
|
Foliar
|
Normal and biotic
stress
|
Improved soil macronutrients, soil properties, and leaf yield
|
Ponmurugan
et al. (2016)
|
Cu
|
Streptomyces capillispiralis
Ca-1
|
-
|
Plate assay
|
Biotic stress
|
Antifungal against Alternaria spp., Aspergillus niger, Pythium spp. and Fusarium spp.
|
Hassan et al.
(2018)
|
Zn
|
Rhizobium
|
Phaseolus vulgaris L.
|
Foliar
|
Normal
|
Increased plant height, leaf area, number of leaves per plant, nutrient uptake, pod yield, and
carbohydrate and protein content in pods
|
Morsy et al.
(2017)
|
ZnO
|
Composted biochar, Farmyard manure
|
Triticum aestivum
|
Foliar
|
Cd stress
|
Increased plant biomass, chlorophyll contents, yield, and activities of peroxidase and superoxide dismutase of wheat while decreased Cd concentrations in shoots, roots, husks, and grains of wheat
|
Bashir et al.
(2020)
|
Ag
|
Nitroxin: Azospirillum
Azotobacter
|
Solanum tuberosum
L
|
Seed priming
|
Normal
|
Improved number of tubers per plant, diameter of tubers, and weight of tubers
|
Davod et al.
(2011)
|
Ti
|
Azotobacter
chroococcum, Azospirillum
brasilense,
Azorhizobium caulinodans
|
Tritico secale
|
Foliar
|
Cd stress
|
Increased chlorophyll content, relative water content, 1000-grain weight, and grain yield
Decreased CAT, SOD, leaf Cd and seed Cd content
|
Ghooshchi (2017)
|
Si nano-biofertilizer (SiNBF)
Silicon is considered as a quasi-essential element for plants (Das et al., 2020). Its use has been shown to: (i) boost photosynthesis in plants through improved mesophyll conductance (Detmann et al., 2012); (ii) assist plants in maintaining water balance during drought stress by intensifying the action of aquaporin through modulation of the expression plasma membrane intrinsic protein (PIP) gene and lowering ROS that cause aquaporin suppression (Chen et al., 2018); (iii) increase metabolite production and improve plant growth (Frew et al., 2018); (iv) act as a potent anti-pathogenic agent against phyto-pathogens like Fusarium oxysporum, Aspergillus niger, Penicillium citrinum, Blumeria graminis, and Magnaporthe grisea (Hernandez-Diaz et al., 2021); and (v) lessen the damaging effects of oxidative stress on plants (Frew et al., 2018, Das et al., 2021).
Si nano-biofertilizers (SiNBFs) improve nutrient (Mn, S, P, and K) translocation from soil to plant, minimize heavy metal uptake and deposition in food crops, and reduce UV stress. SiNBFs with Mesorhizobium sp. and Pseudomonas sp. has been reported to improve plant growth, soil structure, and fertility and have been offered as an inventive Si source with numerous applications, such as improving plant tolerance to biotic and abiotic stresses, wastewater treatment, food processing, nano-additives in the paper industry, biosensors, and biomarkers (Sharma et al., 2022).
Fe nano-biofertilizer (FeNBF)
Iron is an essential micronutrient needed for several plant metabolic processes, including photosynthesis, respiration, DNA synthesis and repair. It serves as both a co-factor and a catalyst in a number of biological processes. Its deficiency prevents the production of chlorophyll, resulting in necrosis and chlorosis in the leaves (Kaur et al., 2022).
The commonly used iron nanoparticles (FeNPs) are iron oxide hydroxide, ferric oxide, ferrous ferric oxide, and iron mineral complex. Application of FeNPs (i) increases the content of phenol, vitamin C and glutathione in Solanum lycopersicum (El-Desouky et al., 2021), (ii) induces the selective uptake of substances from the root membrane and reduces the absorption of sodium, which increases the potassium content in the shoots and makes the plant salt resistant ((El-Desouky et al., 2021), (iii) reduces the toxic effect of ions and stimulates the germination of T. aestivum and increases root and shoot length compared to untreated plants (Yasmeen et al., 2015), and (iv) minimizes the uptake of Cd and increases Fe content in plant grains and tissues, and improves plant growth by suppressing the negative effects of Cd stress on plants (Adrees et al., 2020). The deleterious effects of arsenic contamination in Cucurbita moschata were lessened by the FeNBF of Bacillus subtilis, which increased photosynthesis, gaseous circulation, formation of superoxide dismutase, and catalase (Akhtar et al., 2022).
Cu nano-biofertilizer (CuNBF)
A crucial micronutrient for many enzymes and proteins, Cu plays a function in a number of mitochondrial and chloroplast processes, including iron mobilization, hormone signaling, protein trafficking, mitochondrial respiration, and cell metabolism. Its deficiency causes plant leaf curling, stunting and chlorosis, disruption of photosynthesis, and reduced nitrogen and carbohydrate metabolism (Rehman et al., 2018).
Cu nano-biofertilizers (CuNBFs) can be produced using either an intracellular method (e.g., using the copper-resistant Bacillus cereus) or an extracellular method (e.g., using extracts from various cellular systems, such as the dead biomass of Hypocrea lixii and Trichoderma koningiopsis) (Tiwari et al., 2016). Copper sulfate NBF was synthesized from extracellular metabolites of Penicillium aurantiogriseum, P. citrinum and P. waksmanii. By increasing water status, maintaining photosynthesis rates, enhancing the production of bioactive compounds, catalase and superoxide dismutase activity, and antioxidant activities, CuNBFs can help plants become more tolerant to drought, which in turn encourages crop growth and yield even in stressful environments (Mahapatra et al., 2022).
Zn nano-biofertilizer (ZnNBF)
Zinc, an essential micronutrient, is involved in many biochemical processes, including protein, carbohydrates, and starch metabolism in plants, DNA transcription, photosynthesis, and acting as an enzyme cofactor. It also maintains the structure and functionality of cellular membranes, regulates stomata opening and closing, and affects the potassium level in cells. Zn deficiency prevents tillering, produces chlorosis, stunts crop development, downregulates esterase, prevents pollen synthesis, and slows the rate of fertilization and seed germination (Akhtar et al., 2022).
Zn nano-biofertilizer (ZnNBF) progressively releases nutrients in a controlled manner while having a high translocation in plants. Its application actively contributes to auxin production, improves germination, pollen quality and soil fertility and reduces the negative effects of environmental stressors, improves water and nutrient uptake and preserves membrane integrity (Jamdagni et al., 2018).
Ag nano-biofertilizer (AgNBF)
Silver ions play a crucial role in shoot and root development, somatic embryogenesis, and genetic transformation. Its use lowers ethylene synthesis in plants, lowers the risk of chlorophyll degradation and chlorosis, increases the formation of secondary metabolites, slows the aging process, and boosts crop growth and grain yield (Dawadi et al., 2021).
Silver nanoparticles (AgNPs) have a wider range of antimicrobial properties than their bulk counterparts, providing plants with better protection from pathogens such as fungi and rot. When enhanced with biofertilizers, these properties will help improve plant disease management. AgNBF biosynthesized from fungi has potent antifungal and antibacterial effects against Trichoderma sp., Staphylococcus aureus, Bacillus cereus, and Enterobacter aerogenes at high concentrations (Fatima et al., 2018). AgNPs coated on NBFs act as filters for pathogenic microbes in agroecosystems (Singh et al., 2021). The application of AgNBFs to crops has been shown to promote plant growth even at low concentrations. This is demonstrated by superior root and shoot elongation, high leaf surface area, higher chlorophyll and protein concentration as in Phaseolus vulgaris and Zea mays at various concentrations in comparison to the one without treatments (Akhtar et al., 2022).
Ti nano-biofertilizer (TiNBF)
There is a wide range of applications for titanium nanoparticles (TiO2NPs) in the environmental, industrial, and pharmaceutical sectors (Sharma et al., 2023). Structure, stability, biocompatibility, and customizable hydrophilicity make TiO2NPs an attractive and sustainable solution for ecological and agricultural constraints. It has been demonstrated that green-synthesized TiO2NPs are efficient nanofertilizers that improve photo-catalysis by increasing plant sunlight capturing potential, protect plants from the phytopathogens (e.g., Alternaria brassicae), ameliorate biotic and abiotic stresses in plants, and boost plant biomass yield (Tan et al., 2018). Nevertheless, TiO2NPs have been reported to be hazardous at high doses. Solanum lycopersicum seeds treated with 25 and 50 g/mL concentrations of Trichoderma citrinoviride extract TiO2NPs show favorable results. These myco-produced NBFs have a positive impact on seed germination and seedling health, as well as enhancing chlorophyll, antioxidant mechanisms, and carotenoid levels (Sonawane et al., 2021).
Methods of NBF application, and its uptake and translocation in plants
The application methods of NBFs include soil, foliar, and seed priming (Sharma et al., 2023). A soil application of NBFs can promote plant growth while restoring soil fertility. The activity, mobility, and bio-availability of NBF in soil are severely restricted by heterogeneous aggregation between NPs and soil particles. Foliar spraying of NBF would therefore be a better method for allowing NPs to quickly infiltrate plant tissues and drastically lower reactive oxygen species (ROS) (Bairwa et al., 2023). Seed priming is a pre-sowing procedure that involves soaking seeds in NBFs to accelerate seed germination and seedling production and reduce fertilizer usage. It stimulates plant growth promoting hormones and upregulates stress-resistance genes (Nile et al., 2022).
Nanoparticles adhere to plant surfaces via electrostatic, hydrophobic, and vander Waals forces after coming into contact with root, leaf, and seed surfaces (Khan et al., 2019). In contrast to the leaf, where NPs enter mostly through stomata but also through trichomes, the uptake of NPs by roots happens primarily through physiologically active lateral roots and root hairs. NPs travel upward through xylem (apoplastic transport) and downward through phloem tissues (symplastic transport) after entering roots and leaves. There are various mechanisms through which NPs penetrate plant cells and move intercellularly, such as cell wall pores, endocytosis, plasmodesmata, aquaporins, and ion transporters (Wu et al., 2022). The chemical composition, shape, size, and aggregation state of NPs all play a significant role in the mechanisms that regulate their entry and migration into plant cells. It also depends on the plant species, since the receptors vary from plant to plant. A plant may serve as an excluder for one type of NP and an accumulator for another type (Khan et al., 2019).
Mechanism of NBF mediated plant growth promotion
The synergism of the NP and biofertilizers for promoting plant growth serves as the foundation for the mechanism of NBF-mediated plant growth promotion. NPF promotes plant growth through a wide array of mechanisms, such as (i) increasing nutrient availability and nutrient uptake efficacy of plants, (ii) synthesis of phytohormones (i.e., auxins, gibberellins, cytokinins and abscisic acid), (iii) biocontrol of phytopathogens, (iv) alleviation of biotic and abiotic stresses (such as salinity, drought, flooding, etc.), (v) increasing photosynthate deposition and nutrient transport, and (vi) enhancing photosynthetic activity of plants (Mahapatra et al., 2022; Sharma et al., 2023). The synthesis, characteristics, application methods, and effects of NBF are shown in Figure 1.
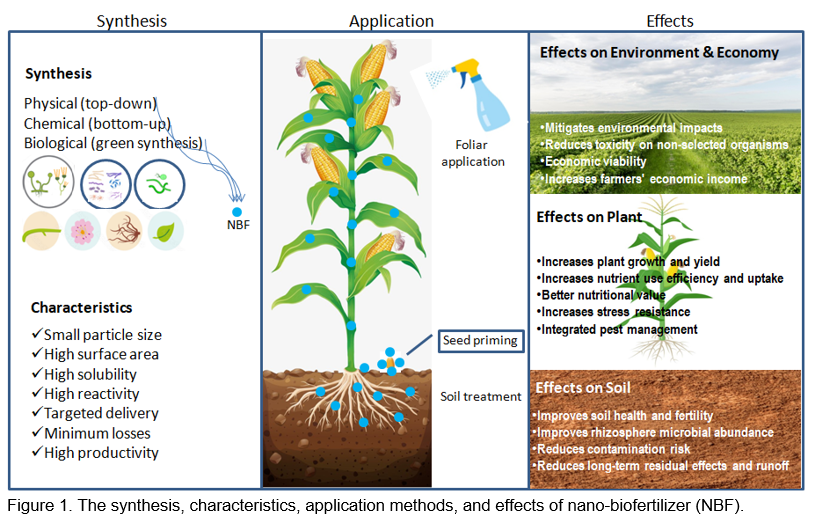
Plant growth promoting microorganisms (PGPM) based NBF has been reported to increase the plant growth and yield by increasing (a) nutrient availability (e.g., N2-fixing microorganisms provide N and phosphorus solubilizing microorganisms provide P to the plant) to the plant, (b) photosynthate deposition and nutrient transport, and (c) phytohormone production. Compared to the crop response reported when NPs were added directly as fertilizer, NBF synthesized by enclosing biofertilizers (such as Pseudomonas fluorescens, Bacillus subtilis, and Paenibacillus elgii) in silver or gold NPs was extremely effective in encouraging plant growth in several different agricultural crops (Sharma et al., 2023). Neem cake with PGPR, a nano-scale fertilizer, can increase agricultural yield in leguminous crops by promoting seedling germination and efficiently delivering nutrients to plants (Mala et al., 2017). Chitosan is a high carbon and nitrogen polymer used for the synthesis of nanocompounds that stimulates and supports the growth of beneficial microbes in NBF. Its application increased inorganic nutrient uptake, net photosynthesis ability via enhanced chlorophyll contents, germination rate, shoots height, and root length of tomato plants (Rendina et al., 2019). NBF containing silicon NP aids in improved photosynthesis of plants by enlarging chloroplasts and increasing grana quantity in leaves. It is also synergistically supported by the angle of leaf opening, leaf uprightness, and reduced self-shading, as seen in rice, wheat, and sugarcane (Frew et al., 2018).
Both biotic and abiotic plant stress have harmful consequences on plants, including disruption of the electron transport chain in mitochondria, a reduction in chlorophyll content in chloroplasts, and DNA damage from ROS. NBF reduces plant stress by upregulating genes responsible for the production of antioxidants, osmolytes and stress-related proteins, reducing the harmful effects of ROS on plants and maintaining cell structure and function. An NBF-induced increase in plant resilience by stimulation of pathogenesis-associated proteins (e.g., catalase, peroxidase, polyphenol oxidase, glucanase, chitinase), and nonenzymatic antioxidants (e.g., phenols and flavonoids), has been reported (Dong et al., 2022). Chitosan immobilized silica nanocomposites and chitosan were used to make a NBF containing Glomus mossease, Trichoderma viridae and Bacillus subtilis that could inhibit tomato bacterial wilt caused by Ralstonia solanacearum (Rendina et al., 2019).
Constraints and future perspective
Nano-biofertilizers may have adverse ecological and/or toxicological effects. Crop growth and development may be adversely affected by non-targeted cell death or apoptosis caused by ROS generation and NBFs' unfavorable involvement in cellular metabolism (Wu et al., 2022). These consequences for crops are likely if we are ignorant about the dosage response and mortality of NPs in biological systems. Toxic effects of NBFs can be minimized by using dose metrics that measure particle charge, concentration (number or mass), surface area, and net size as benchmarks for quantifying NP toxicity (Mahapatra et al., 2022). Some NPs used in the formulation of NBF may adversely affect beneficial plant-associated microbes, thereby affecting plant growth and yield. For example, AgNPs have been shown to have a potential antibacterial effect on soil microbial growth at concentrations lower than other heavy metals. Studies have shown that AgNPs can have toxic effects on beneficial microbial communities, including nitrogen-fixing bacteria, ammonifying bacteria, and chemolithotrophic bacteria, negatively affecting plant growth and yield (Bahrulolum et al., 2021). Therefore, it is necessary to select the compatible NPs and biofertilizers and combine NPs and biofertilizers appropriately to produce an efficient, eco- friendly NBF. Although post-nano-based biofertilizer formulations can be used to study ecotoxicological aspects and threats to safety and sanitation for various NBFs, including organic and green NBFs, there is still a high expectation for these nanocommodities to be used throughout the entire agricultural supply chain, including processing, packaging, preservation, transportation, storage, and final delivery.
By offering useful and innovative solutions, nanotechnology is actively participating in tackling a variety of environmental issues. While the field of biomedical sciences has lately improved diagnostic and therapeutic techniques due to phenomenal advancements in nanotechnology, knowledge of the interactions between plants and NBFs is still in its infancy stage. The formulation and application of eco-friendly NBF has received little research so far. Future studies on the parameters below may enable NBF to serve agriculture and transform into a cutting-edge tool for the advancement of sustainable agricultural development.
- It is necessary to create a multipurpose NBF that is suitable for many crops.
- To create NBF with superior quality, a longer shelf life, a cheap cost, and convenience of use, it is necessary to combine cutting-edge technology, protocols, machine learning, and artificial intelligence (AI).
- NBF needs to undergo a cost-benefit analysis, and its compatibility with various soil and environmental conditions needs to be tested.
- NBF should be thoroughly examined to determine their effects on environments and human health.
- The impact of NBF on plants’ physicochemical, biochemical, and molecular systems at the cellular and molecular levels under both ambient and stress conditions requires more investigation.
- Investigation into the long-term life cycle effects of NBFs in the agricultural ecosystem is necessary.
- Farmers must be educated on the drawbacks of chemical fertilizer as well as how NBFs will reduce costs and provide long-term benefits.
CONCLUSIONS
With improved overall plant growth dynamics, targeted nutrient delivery, soil fertility, and induced systemic resistance than either NPs or biofertilizers alone, NBFs can pave a new path in crop sustainability. Agriculture would benefit greatly from the use of NBFs, so they must be produced on an industrial scale with stable formulations and environmentally friendly processes. A great deal of effort is being put into utilizing natural resources and implementing biological synthesis methods with several proven advantages, including being environmentally friendly, easy to scale up, and cost-effective; this makes the green synthesis of NBFs an exciting endeavor. There is still a lack of knowledge about the underlying mechanisms that govern the synthesis, action, and stabilization of green synthesized NBFs. To meet the need for NBFs in agriculture, a deeper investigation of biological nanofactories is prompted by the abundance of microorganisms and plants that have been effectively exploited for the synthesis of green NBFs. Research on the bioavailability and biocompatibility of NBF is still in its early phases, and much more needs to be done in this area. A prosperous agro-economy can be established based on NBFs because they can provide favorable conditions for enhanced productivity, improved resource conservation, and a healthier environment under climate change.
REFERENCES
Adrees, M., Khan, Z.S., Ali, S., Hafeez, M., Khalid, S., Rehman, M.Z.U., Hussain, A., Hussain, K., Chatha, S.A.S. and Rizwan, M., 2020. Simultaneous mitigation of cadmium and drought stress in wheat by soil application of iron nanoparticles. Chemosphere 238: 124681.
Akhtar, N. and Ilyas, N., 2022. Role of nanosilicab to boost the activities of metabolites in Triticum aestivum facing drought stress. Plant Soil 1–17.
Akhtar, N., Ilyas, N., Meraj, T.A., Pour-Aboughadareh, A., Sayyed, R.Z., Mashwani, Z.U.R. and Poczai, P., 2022. Improvement of plant responses by Nano-bio-fertilizer: a step towards sustainable agriculture. Nanomaterials 12 (6): 965.
Bahrulolum, H., Nooraei, S., Javanshir, N., Tarrahimofrad, H., Mirbagheri, V.S., Easton, A.J. and Ahmadian, G., 2021. Green synthesis of metal nanoparticles using microorganisms and their application in the agrifood sector. J. Nanobiotechnol. 19(1): 1-26.
Bairwa, P., Kumar, N., Devra, V. and Abd-Elsalam, K.A., 2023. Nano-Biofertilizers Synthesis and Applications in Agroecosystems. Agrochemicals, 2(1): 118-134.
Baragaño, D., Forján, R., Fernández, B., Ayala, J., Afif, E. and Gallego, J.L.R., 2020. Application of biochar, compost and ZVI nanoparticles for the remediation of As, Cu, Pb and Zn polluted soil. Environ. Sci. Pollut. Res. 27: 33681–33691.
Bashir, A., Rizwan, M., Ali, S., Adrees, M. and Qayyum, M.F., 2020. Effect of composted organic amendments and zinc oxide nanoparticles on growth and cadmium accumulation by wheat; a life cycle study. Environ. Sci. Pollut. Res. 27 (19): 23926–23936.
Chen, D., Wang, S., Yin, L. and Deng, X., 2018. How does silicon mediate plant water uptake and loss under water deficiency? Front. Plant Sci. 9: 281.
Daryabeigi Zand, A., Tabrizi, A.M. and Heir, A.V., 2020. The influence of association of plant growth promoting rhizobacteria and zero-valent iron nanoparticles on removal of antimony from soil by Trifolium repens. Environ. Sci. Pollut. Res. 27 (34): 42815–42829.
Das, S., Galgo, S.J., Alam, M.A., Lee, J.G., Hwang, H.Y., Lee, C.H. and Kim, P.J., 2020. Recycling of ferrous slag in agriculture: Potentials and challenges. Cri. Rev. Environ. Sci. Technol. 52(8): pp.1247-1281.
Das, S., Kim, G.W., Lee, J.G., Bhuiyan, M.S.I. and Kim, P.J., 2021. Silicate fertilization improves microbial functional potentials for stress tolerance in arsenic-enriched rice cropping systems. J. Hazard. Mater. 417: 125953.
Davod, T., Reza, Z., Ali, V.A. and Mehrdad, C., 2011. Effects of nanosilver and nitroxin biofertilizer on yield and yield components of potato minitubers. Int. J. Agric. Biol. 13 (6): 986–990.
Dawadi, S., Katuwal, S., Gupta, A., Lamichhane, U., Thapa, R., Jaisi, S., and Parajuli, N., 2021. Current research on silver nano-particles: synthesis, characterization and applications. J. Nanomater. 2021.
de França Bettencourt, G.M., Degenhardt, J., Torres, L.A.Z., de Andrade Tanobe, V.O. and Soccol, C.R., 2020. Green biosynthesis of single and bimetallic nanoparticles of iron and manganese using bacterial auxin complex to act as plant bio-fertilizer. Biocatal. Agric. Biotechnol. 30: 101822.
Detmann, K.C., Araujo, W.L., Martins, S.C., Sanglard, L.M., Reis, J.V., Detmann, E., Rodrigues, F.A., Nunes-Nesi, A., Fernie, A.R. and DaMatta, F.M., 2012. Silicon nutrition increases grain yield, which, in turn, exerts a feed-forward stimulation of photosynthetic rates via enhanced mesophyll conductance and alters primary metabolism in rice. New Phytol. 196 (3): 752–762.
Dong, B.R., Jiang, R., Chen, J.F., Xiao, Y., Lv, Z.Y. and Chen, W.S., 2022. Strategic nanoparticle mediated plant disease resistance. Crit. Rev. Biotechnol. 1–16.
El-Desouky, H.S., Islam, K.R., Bergefurd, B., Gao, G., Harker, T., Abd-El-Dayem, H. and Zewail, R.M., 2021. Nano iron fertilization significantly increases tomato yield by increasing plants'vegetable growth and photosynthetic efficiency. J. Plant Nutr. 44 (11): 1649–1663.
Fatima, F., Pathak, N., Verma, S.R. and Bajpai, P., 2018. Toxicity and immunomodulatory efficacy of biosynthesized silver myconanosomes on pathogenic microbes and macrophage cells. Artif. Cells Nanomed. Biotechnol. 46 (8): 1637–1645.
Frew, A., Weston, L.A., Reynolds, O.L. and Gurr, G.M., 2018. The role of silicon in plant biology: a paradigm shift in research approach. Ann. Bot. 121(7): 1265-1273.
Ghooshchi, F., 2017. Influence of titanium and bio-fertilizers on some agronomic and physiological attributes of triticale exposed to cadmium stress. Glob. NestJ. 19 (3): 458–463.
Hassan, S.E.D., Salem, S.S., Fouda, A., Awad, M.A., El-Gamal, M.S. and Abdo, A.M., 2018. New approach for antimicrobial activity and bio-control of various pathogens by biosynthesized copper nanoparticles using endophytic actinomycetes. J. Radiat. Res. Appl. Sci. 11 (3): 262–270.
Hatami, M., Khanizadeh, P., Bovand, F. and Aghaee, A., 2021. Silicon nanoparticle mediated seed priming and Pseudomonas spp. inoculation augment growth, physiology and antioxidant metabolic status in Melissa officinalis L. plants. Ind. Crops Prod. 162: 113238.
Hernandez-Diaz, J.A., Garza-Garcia, J.J., Zamudio-Ojeda, A., Leon-Morales, J.M., Lopez-Velazquez, J.C. and Garcia-Morales, S., 2021. Plant mediated synthesis of nanoparticles and their antimicrobial activity against phytopathogens. J. Sci. Food Agric. 101 (4): 1270–1287.
Jamdagni, P., Rana, J.S. and Khatri, P., 2018. Comparative study of antifungal effect of green and chemically synthesised silver nanoparticles in combination with carbendazim, mancozeb, and thiram. IET Nanobiotechnol. 12 (8): 1102–1107
Jamkhande, P.G., Ghule, N.W., Bamer, A.H. and Kalaskar, M.G., 2019. Metal nano-particles synthesis: an overview on methods of preparation, advantages and disadvantages and applications. J. Drug Deliv. Sci. Technol. 53: 101174.
Kah, M., Kookana, R.S., Gogos, A. and Bucheli, T.D., 2018. A critical evaluation of nanopesticides and nanofertilizers against their conventional analogues. Nat. Nanotechnol. 13(8): 677-684.
Kah, M., Tufenkji, N. and White, J.C., 2019. Nano-enabled strategies to enhance crop nutrition and protection. Nat. Nanotechnol. 14(6): 532-540.
Kaur, H., Kaur, H., Kaur, H. and Srivastava, S., 2022. The beneficial roles of trace and ultratrace elements in plants. Plant Growth Regul. 1–18.
Khan, M.R., Adam, V., Rizvi, T.F., Zhang, B., Ahamad, F., Jośko, I., Zhu, Y., Yang, M. and Mao, C., 2019. Nanoparticle–plant interactions: two‐way traffic. Small, 15(37): 1901794.
Kumar, S., Sindhu, S.S. and Kumar, R., 2022. Biofertilizers: An ecofriendly technology for nutrient recycling and environmental sustainability. Curr. Res. Microb. Sci. 3: 100094.
Mahapatra, D.M., Satapathy, K.C. and Panda, B., 2022. Biofertilizers and nano-fertilizers for sustainable agriculture: phycoprospects and challenges. Sci. Total Environ. 803: 149990.
Mala, R., Celsia Arul Selvaraj, R., Barathi Sundaram, V., Blessina Siva Shanmuga Rajan, R. and Maheswari Gurusamy, U., 2017. Evaluation of nano structured slow release fertilizer on the soil fertility, yield and nutritional profile of Vigna radiata. Rec. Pat. Nanotechnol. 11 (1): 50–62.
Morsy, N.M., Shams, A.S. and Abdel-Salam, M.A., 2017. Zinc foliar spray on snap beans using nano-Zn with N-soil application using mineral, organic and biofertilizer. Middle East J. Agric. Res. 6 (4): 1301–1312.
Nile, S.H., Thiruvengadam, M., Wang, Y., Samynathan, R., Shariati, M.A., Rebezov, M. and Kai, G., 2022. Nano-priming as emerging seed priming technology for sustainable agriculture recent developments and future perspectives. J. Nanobiotechnol. 20 (1): 1–31.
Ponmurugan, P., Manjukarunambika, K., Elango, V. and Gnanamangai, B.M., 2016. Antifungal activity of bio-synthesised copper nanoparticles evaluated against red root rot disease in tea plants. J. Exp. Nanosci. 11 (13): 1019–1031.
Rehman, M., Liu, L., Wang, Q., Saleem, M.H., Bashir, S., Ullah, S. and Peng, D., 2019. Copper environmental toxicology, recent advances and future outlook: a review. Environ. Sci. Pollut. Res. 26 (18): 18003–18016.
Rendina, N., Nuzzaci, M., Scopa, A., Cuypers, A. and Sofo, A., 2019. Chitosan elicited defense responses in cucumbermosaic virus (CMV) infected tomato plants. J. Plant Physiol. 234: 9–17.
Sharma, B., Tiwari, S., Kumawat, K.C. and Cardinale, M., 2023. Nano-biofertilizers as bio-emerging strategies for sustainable agriculture development: Potentiality and their limitations. Sci. Tot.Environ. 860: 160476.
Singh, P., Kim, Y.J., Zhang, D. and Yang, D.C., 2016. Biological synthesis of nanoparticles from plants and microorganisms. Trends Biotechnol. 34(7): 588-599.
Singh, R.P., Handa, R., and Manchanda, G., 2021. Nanoparticles in sustainable agriculture: an emerging opportunity. J. Control. Release 329: 1234–1248.
Sonawane, H., Arya, S., Math, S. and Shelke, D., 2021. Myco-synthesized silver and titanium oxide nanoparticles as seed priming agents to promote seed germination and seedling growth of Solanum lycopersicum: a comparative study. Int. Nano Lett. 11 (4): 371–379.
Sonawane, H., Shelke, D., Chambhare, M., Dixit, N., Math, S., Sen, S., Borah, S.N., Islam, N.F., Joshi, S.J., Yousaf, B. and Rinklebe, J., 2022. Fungi-derived agriculturally important nanoparticles and their application in crop stress management–Prospects and environmental risks. Environ. Res. 212: 113543.
Tan, W., Peralta-Videa, J.R. and Gardea-Torresdey, J.L., 2018. Interaction of titanium dioxide nanoparticles with soil components and plants: current knowledge and future research needs: a critical review. Environ. Sci. Nano 5 (2): 257–278.
Tiwari, M., Jain, P., Hariharapura, R.C., Narayanan, K., Bhat, U., Udupa, N. and Rao, J.V., 2016. Biosynthesis of copper nanoparticles using copper-resistant Bacillus cereus, a soil isolate. Process Biochem. 51 (10): 1348–1356.
Wu, H. and Li, Z., 2022. Nano-enabled agriculture: how nanoparticles cross barriers in plants?. Plant Communications.
Yasmeen, F., Razzaq, A., Iqbal, M.N. and Jhanzab, H.M., 2015. Effect of silver, copper and iron nanoparticles on wheat germination. Int. J. Biosci. 6: 112–117.
Nano-biofertilizer for Sustainable Agriculture
DOI: https://doi.org/10.56669/CDMA8114
ABSTRACT
Modern cropping systems rely heavily on chemical or synthetic fertilizers to increase crop yields, but their long-term and continuous application can have detrimental effects on the environment, soil biota, and fertility. These issues emphasize the need for highly efficient, cost-effective fertilizers that can increase yields while minimizing environmental damage. As a non-toxic and eco-friendly alternative, bio- and nano-fertilizers are preferred over synthetic (chemical) fertilizers to ensure biosafety in agriculture. In recent years, the use of a combination of nanoparticles and biofertilizer, referred to as nano-biofertilizer (biofertilizer coated with nanomaterial) has been promoted to combine the synergism of individuals for sustainable crop production. While the use of nano-biofertilizer is currently in its infancy phase in agriculture, it has the potential to transform traditional agriculture into smart one. In this review, we provide a comprehensive overview of nano-biofertilizer formulation, characterization, and application, and elucidate the underlying mechanisms by which they promote plant growth and how they help plants cope with biotic and abiotic stresses caused by climate change. We summarize the latest advances of nano-biofertilizer applications in precision agriculture. The limitations and future strategies for formulating effective nano-biofertilizer are discussed.
Keywords: nanoparticles, biofertilizers, plant growth promotion, phytohormones, and biocontrol of phytopathogens
INTRODUCTION
Since the green revolution, agrochemicals (chemical fertilizers and pesticides) have become an indispensable part of intensive cropping systems. Although agrochemicals boost crop yields, their persistent and prolonged use has been shown to have detrimental effects on future agricultural prospects, causing environmental pollution, including greenhouse gas emissions and global warming, soil acidification and degradation, eutrophication of water, loss of biodiversity, emergence of pests, nutrient loss and its biomagnification in food chains, which can pose a health threat (Kah et al., 2019). The exploration of alternative nutrient solutions and modification of existing nutrient sources are therefore crucial for ensuring agricultural sustainability of crop production.
Over the past decade, a great deal of effort has been put into replacing chemical fertilizers with better eco-friendly and cost-effective alternatives. A promising alternative to chemical fertilizers for sustainable agriculture is using plant growth promoting rhizobacteria (PGPRs), endo- and ectomycorrhizal fungi, cyanobacteria and many other useful microscopic organisms as biofertilizers (Kumar et al., 2022). The increased availability of plant nutrients (e.g., N, P, K, and Zn), the modulation of phytohormones (e.g., indoleacetic acid and gibberellic acid), the biocontrol of phytopathogens, and the amelioration of biotic and abiotic stresses are some of the mechanisms by which plant growth and yield are improved in response to biofertilizer inoculation (Kumar et al., 2022). Biofertilizers, however, present a number of challenges, such as poor shelf-life, on-field stability, performance under fluctuating climatic conditions, and the most significant issue is high dosage requirements. In recent years, nanotechnology has opened up unprecedented possibilities for agricultural sustainability and intensive production practices through the development of nanofertilizers and nanopesticides (Kah et al., 2018). Owing to its distinct characteristics such as high surface area, high solubility, and lightness, nanoparticle (NP)-based formulation has embraced many implementation domains in agriculture, such as improved nutrient use efficiency, enhanced plant growth, and disease resistance, making it preferable compared to synthetic fertilizers and biofertilizers (Kah et al., 2018, 2019). By integrating nanotechnology and biotechnology, modern agriculture strives to make farming more efficient, cost-effective, and eco-sustainable while ensuring food security. Nano-biofertilizer (NBF), a hybrid of nanoparticle (NP) and biofertilizer that produces a more potent option for eco-sustainable agriculture, represents an advancement in agricultural innovation (Sharma et al., 2023). NBFs are made by encapsulating biofertilizers reduced to nanoscale (1-100 nm) within nanomaterial coatings. It overcomes the drawbacks of biofertilizers, reduces the dependency on chemical fertilizers, and combats major issues of crop production, food security, sustainability and eco-safety. NBFs provide a number of important advantages, including: (i) slow and steady release of nutrient to crop plants without any unintended loss; (ii) increased nutrient use efficiency; (iii) enriched beneficial microorganisms in soils; (iv) improved disease resistance of crops; (v) mass production in less time; (vi) cost effectiveness; and (vii) renewable fertilization (Sharma et al., 2023). Yet, the application of NBF is advancing from the experimental to the practical realms. Due to the limited understanding of NBF synthesis, especially the green synthesis of NBFs, their effective application methods, and the mechanisms by which NBFs mediate plant growth, they have not been widely endorsed and commercialized. This review article explores the formulation, characterization, and application of NBFs, as well as unraveling the mechanisms by which they promote plant growth and cope with climate change-induced stresses. We provide a summary of the most recent developments in the use of NBFs in precision agriculture. The disadvantages and potential approaches for developing effective NBFs are discussed.
Formulation and characterization of NBFs
Nano-biofertilizer formulation mainly involves top-down and bottom-up approaches. In top-down approach, NBFs are produced from large-sized raw components, whose size is reduced by various mechano-physical procedures, whereas bottom-up approaches develop NPs from atoms, compounds, or smaller particles through chemical and biological reactions. A wide variety of mechano-physicochemical techniques, such as mechanical grinding/milling, co-precipitation, chemical etching, sputtering, lithography, spray pyrolysis, laser pyrolysis, evaporation-condensation, sol-gel, photochemical reduction, and laser ablation techniques have been used in top-down and bottom-up approaches for the synthesis of metal NPs (Jamkhande et al., 2019). In general, NBFs are synthesized by physicochemical methods and biological methods (bottom-up), although the latter has emerged as a fast, measurable, non-toxic, energy efficient and economically viable method (Sharma et al., 2023).
In biological methods for NP synthesis, microorganisms are commonly used because they release inorganic materials either intracellularly or extracellularly, such as magnetostatic bacteria that release magnetite, diatoms that release silicious materials, and S-layer bacteria that release calcium carbonate and gypsum (Singh et al., 2016). For the intracellular synthesis of NPs, microorganisms take metal ions from the surrounding environment and convert them to elemental forms using enzyme reduction, while for the extracellular synthesis of NPs, microorganisms produce proteins and enzymes that reduce metal ions and make them stable (Bahrulolum et al., 2021). In general, biological methods offer more control and management over crystal shape, size, composition, and structural stabilization of NP while putting an end to the requirement for reactive and toxic reducing chemicals, which are hazardous to microorganisms (Bahrulolum et al., 2021).
In recent years, the green synthesis of NBFs has emerged as an efficient, eco-friendly, and economically feasible method with a number of advantages over conventionally produced NBFs (Bairwa et al., 2023). Green NBFs are synthesized using biogenic and microbial compounds such as plant (stem, root, seed, leaf, fruit, flower) extracts, fermented extracts, enzymes, organic polymers (extracellular polymeric substance) and other polysaccharides as reducing agents in bionanofactories where metal ions are reduced to create biocompatible nanocomposites. A wide range of microorganisms, such as plant beneficial-bacteria like Clostridium thermoaceticum, Desulfovibrio desulfuricans, Klebsiella aerogenes, cyanobacteria like Spirulina, Nostoc, Anabaena, Synechocystis, Phormidium, Lyngbya, Oscillatoria and green algae like Chlorella, Scenedesmus, Chlorococcum, Cosmarium, Chlamydomonas, Botryococcus have been used as potential biofactories to synthesize a variety of metal NPs containing metals like silver, gold, copper, zinc, titanium, palladium, and nickel in an economical and environmentally friendly way (Bairwa et al., 2023; Sharma et al., 2023). Recently, myconanotechnology has emerged as a promising research area in which fungi (e.g., Aspergillus sp., Fusarium oxysporum) can be used to synthesize metal NPs both intracellularly and extracellularly. Due to their greater efficacy and widespread distribution in nature, fungi have been chosen over other microorganisms for the synthesis of metal NPs (Sonawane et al., 2022).
The synthesis of NBF involves three important steps: (1) the growth of the biofertilizer culture; (2) its encapsulation with NPs; and (3) evaluation and assessment of its performance, quality, purity, and self-life (Bairwa et al., 2023). For the production of fungal NBF, MGYP broth (maltose, glucose, yeast potato) was used to culture the fungus (Alternaria brassicae) for 5 days at 27 °C and the fungal colonies were recovered by sieving. By adding AgNO3 to harvested biomass and incubating in the dark, silver-nanoparticles (AgNPs) were produced. A UV–VIS spectrophotometer (350–650 nm) was used to test and validate the produced AgNPs, which were then freeze-dried and stored for use in the future (Fatima et al., 2018). NBFs are increasingly being synthesized using cell-free supernatant from bacteria. It can also be synthesized in the form of microcapsule. For this, plant growth promoting rhizobacteria (PGPR) suspension was mixed with 1.5% sodium alginate, 3% starch, and 4% bentonite in a 2:1 ratio. After the mixture was coated with calcium chloride solution, the microcapsules were washed with sterile distilled water. Mono- and bimetallic iron and manganese NPs were successfully synthesized using the endophyte bacterium Paenibacillus polymyxa supernatant solution with auxin (IAA) (de França Bettencourt et al., 2020). Several factors, including microbial origin, reaction temperature, pH, pressure, incubation time, and metal salt concentration, control the synthesis of NBFs.
Nanoparticles used in the synthesis of NBFs
Nanoparticles commonly used for NBFs formulation are silicon (Si), iron (Fe), copper (Cu), zinc (Zn), silver (Ag), and titanium (Ti). The mechanisms through which various NPs enhance plant growth vary. The constituents of NBFs (NPs + biofertilizer), targeted plant, mode of application, and response of plants to NBFs are shown in Table 1.
Table 1. The constituents of nano-biofertilizer (NBF), targeted plant, mode of application, and response of plants to NBFs
Nanobiofertilizer
Targeted plant
Mode of application
Condition
Plant responses
References
Nano particle
Biological component
Si
Pseudomonas fluorescens,
P. putida
Melissa officinalis L.
Seed priming
Normal
Increased photosynthetic pigments, total soluble protein and phenolic content, leaf water content, and plant biomass
Hatami et al.
(2021)
SiO2
Azospirillum brasilense,
Bacillus sp., Azospirillum
lipoferum
Triticum aestivum
Soil
Drought
Increased photosynthetic pigments, osmolytes content, relative water content, membrane stability index, phenol, and flavonoid content, indole acetic acid and cytokinin content, germination percentage, germination index, and yield
Akhtar and Ilyas
(2022)
Fe (zero-valent)
Compost and biochar
Brassica juncea L.
Soil
Heavy metal
stress
Enhanced soil cation exchange capacity, soil pH, total nitrogen and total carbon, and plant biomass
Baragano et al.
(2020)
Fe (zero-valent)
Pseudomonas
fluorescens
Trifolium repens
Soil
Antimony stress
Improved plant growth and phytoremediation
potential
Daryabeigi Zand
et al. (2020)
Cu
Streptomyces griseus
Tea
Foliar
Normal and biotic
stress
Improved soil macronutrients, soil properties, and leaf yield
Ponmurugan
et al. (2016)
Cu
Streptomyces capillispiralis
Ca-1
-
Plate assay
Biotic stress
Antifungal against Alternaria spp., Aspergillus niger, Pythium spp. and Fusarium spp.
Hassan et al.
(2018)
Zn
Rhizobium
Phaseolus vulgaris L.
Foliar
Normal
Increased plant height, leaf area, number of leaves per plant, nutrient uptake, pod yield, and
carbohydrate and protein content in pods
Morsy et al.
(2017)
ZnO
Composted biochar, Farmyard manure
Triticum aestivum
Foliar
Cd stress
Increased plant biomass, chlorophyll contents, yield, and activities of peroxidase and superoxide dismutase of wheat while decreased Cd concentrations in shoots, roots, husks, and grains of wheat
Bashir et al.
(2020)
Ag
Nitroxin: Azospirillum
Azotobacter
Solanum tuberosum
L
Seed priming
Normal
Improved number of tubers per plant, diameter of tubers, and weight of tubers
Davod et al.
(2011)
Ti
Azotobacter
chroococcum, Azospirillum
brasilense,
Azorhizobium caulinodans
Tritico secale
Foliar
Cd stress
Increased chlorophyll content, relative water content, 1000-grain weight, and grain yield
Decreased CAT, SOD, leaf Cd and seed Cd content
Ghooshchi (2017)
Si nano-biofertilizer (SiNBF)
Silicon is considered as a quasi-essential element for plants (Das et al., 2020). Its use has been shown to: (i) boost photosynthesis in plants through improved mesophyll conductance (Detmann et al., 2012); (ii) assist plants in maintaining water balance during drought stress by intensifying the action of aquaporin through modulation of the expression plasma membrane intrinsic protein (PIP) gene and lowering ROS that cause aquaporin suppression (Chen et al., 2018); (iii) increase metabolite production and improve plant growth (Frew et al., 2018); (iv) act as a potent anti-pathogenic agent against phyto-pathogens like Fusarium oxysporum, Aspergillus niger, Penicillium citrinum, Blumeria graminis, and Magnaporthe grisea (Hernandez-Diaz et al., 2021); and (v) lessen the damaging effects of oxidative stress on plants (Frew et al., 2018, Das et al., 2021).
Si nano-biofertilizers (SiNBFs) improve nutrient (Mn, S, P, and K) translocation from soil to plant, minimize heavy metal uptake and deposition in food crops, and reduce UV stress. SiNBFs with Mesorhizobium sp. and Pseudomonas sp. has been reported to improve plant growth, soil structure, and fertility and have been offered as an inventive Si source with numerous applications, such as improving plant tolerance to biotic and abiotic stresses, wastewater treatment, food processing, nano-additives in the paper industry, biosensors, and biomarkers (Sharma et al., 2022).
Fe nano-biofertilizer (FeNBF)
Iron is an essential micronutrient needed for several plant metabolic processes, including photosynthesis, respiration, DNA synthesis and repair. It serves as both a co-factor and a catalyst in a number of biological processes. Its deficiency prevents the production of chlorophyll, resulting in necrosis and chlorosis in the leaves (Kaur et al., 2022).
The commonly used iron nanoparticles (FeNPs) are iron oxide hydroxide, ferric oxide, ferrous ferric oxide, and iron mineral complex. Application of FeNPs (i) increases the content of phenol, vitamin C and glutathione in Solanum lycopersicum (El-Desouky et al., 2021), (ii) induces the selective uptake of substances from the root membrane and reduces the absorption of sodium, which increases the potassium content in the shoots and makes the plant salt resistant ((El-Desouky et al., 2021), (iii) reduces the toxic effect of ions and stimulates the germination of T. aestivum and increases root and shoot length compared to untreated plants (Yasmeen et al., 2015), and (iv) minimizes the uptake of Cd and increases Fe content in plant grains and tissues, and improves plant growth by suppressing the negative effects of Cd stress on plants (Adrees et al., 2020). The deleterious effects of arsenic contamination in Cucurbita moschata were lessened by the FeNBF of Bacillus subtilis, which increased photosynthesis, gaseous circulation, formation of superoxide dismutase, and catalase (Akhtar et al., 2022).
Cu nano-biofertilizer (CuNBF)
A crucial micronutrient for many enzymes and proteins, Cu plays a function in a number of mitochondrial and chloroplast processes, including iron mobilization, hormone signaling, protein trafficking, mitochondrial respiration, and cell metabolism. Its deficiency causes plant leaf curling, stunting and chlorosis, disruption of photosynthesis, and reduced nitrogen and carbohydrate metabolism (Rehman et al., 2018).
Cu nano-biofertilizers (CuNBFs) can be produced using either an intracellular method (e.g., using the copper-resistant Bacillus cereus) or an extracellular method (e.g., using extracts from various cellular systems, such as the dead biomass of Hypocrea lixii and Trichoderma koningiopsis) (Tiwari et al., 2016). Copper sulfate NBF was synthesized from extracellular metabolites of Penicillium aurantiogriseum, P. citrinum and P. waksmanii. By increasing water status, maintaining photosynthesis rates, enhancing the production of bioactive compounds, catalase and superoxide dismutase activity, and antioxidant activities, CuNBFs can help plants become more tolerant to drought, which in turn encourages crop growth and yield even in stressful environments (Mahapatra et al., 2022).
Zn nano-biofertilizer (ZnNBF)
Zinc, an essential micronutrient, is involved in many biochemical processes, including protein, carbohydrates, and starch metabolism in plants, DNA transcription, photosynthesis, and acting as an enzyme cofactor. It also maintains the structure and functionality of cellular membranes, regulates stomata opening and closing, and affects the potassium level in cells. Zn deficiency prevents tillering, produces chlorosis, stunts crop development, downregulates esterase, prevents pollen synthesis, and slows the rate of fertilization and seed germination (Akhtar et al., 2022).
Zn nano-biofertilizer (ZnNBF) progressively releases nutrients in a controlled manner while having a high translocation in plants. Its application actively contributes to auxin production, improves germination, pollen quality and soil fertility and reduces the negative effects of environmental stressors, improves water and nutrient uptake and preserves membrane integrity (Jamdagni et al., 2018).
Ag nano-biofertilizer (AgNBF)
Silver ions play a crucial role in shoot and root development, somatic embryogenesis, and genetic transformation. Its use lowers ethylene synthesis in plants, lowers the risk of chlorophyll degradation and chlorosis, increases the formation of secondary metabolites, slows the aging process, and boosts crop growth and grain yield (Dawadi et al., 2021).
Silver nanoparticles (AgNPs) have a wider range of antimicrobial properties than their bulk counterparts, providing plants with better protection from pathogens such as fungi and rot. When enhanced with biofertilizers, these properties will help improve plant disease management. AgNBF biosynthesized from fungi has potent antifungal and antibacterial effects against Trichoderma sp., Staphylococcus aureus, Bacillus cereus, and Enterobacter aerogenes at high concentrations (Fatima et al., 2018). AgNPs coated on NBFs act as filters for pathogenic microbes in agroecosystems (Singh et al., 2021). The application of AgNBFs to crops has been shown to promote plant growth even at low concentrations. This is demonstrated by superior root and shoot elongation, high leaf surface area, higher chlorophyll and protein concentration as in Phaseolus vulgaris and Zea mays at various concentrations in comparison to the one without treatments (Akhtar et al., 2022).
Ti nano-biofertilizer (TiNBF)
There is a wide range of applications for titanium nanoparticles (TiO2NPs) in the environmental, industrial, and pharmaceutical sectors (Sharma et al., 2023). Structure, stability, biocompatibility, and customizable hydrophilicity make TiO2NPs an attractive and sustainable solution for ecological and agricultural constraints. It has been demonstrated that green-synthesized TiO2NPs are efficient nanofertilizers that improve photo-catalysis by increasing plant sunlight capturing potential, protect plants from the phytopathogens (e.g., Alternaria brassicae), ameliorate biotic and abiotic stresses in plants, and boost plant biomass yield (Tan et al., 2018). Nevertheless, TiO2NPs have been reported to be hazardous at high doses. Solanum lycopersicum seeds treated with 25 and 50 g/mL concentrations of Trichoderma citrinoviride extract TiO2NPs show favorable results. These myco-produced NBFs have a positive impact on seed germination and seedling health, as well as enhancing chlorophyll, antioxidant mechanisms, and carotenoid levels (Sonawane et al., 2021).
Methods of NBF application, and its uptake and translocation in plants
The application methods of NBFs include soil, foliar, and seed priming (Sharma et al., 2023). A soil application of NBFs can promote plant growth while restoring soil fertility. The activity, mobility, and bio-availability of NBF in soil are severely restricted by heterogeneous aggregation between NPs and soil particles. Foliar spraying of NBF would therefore be a better method for allowing NPs to quickly infiltrate plant tissues and drastically lower reactive oxygen species (ROS) (Bairwa et al., 2023). Seed priming is a pre-sowing procedure that involves soaking seeds in NBFs to accelerate seed germination and seedling production and reduce fertilizer usage. It stimulates plant growth promoting hormones and upregulates stress-resistance genes (Nile et al., 2022).
Nanoparticles adhere to plant surfaces via electrostatic, hydrophobic, and vander Waals forces after coming into contact with root, leaf, and seed surfaces (Khan et al., 2019). In contrast to the leaf, where NPs enter mostly through stomata but also through trichomes, the uptake of NPs by roots happens primarily through physiologically active lateral roots and root hairs. NPs travel upward through xylem (apoplastic transport) and downward through phloem tissues (symplastic transport) after entering roots and leaves. There are various mechanisms through which NPs penetrate plant cells and move intercellularly, such as cell wall pores, endocytosis, plasmodesmata, aquaporins, and ion transporters (Wu et al., 2022). The chemical composition, shape, size, and aggregation state of NPs all play a significant role in the mechanisms that regulate their entry and migration into plant cells. It also depends on the plant species, since the receptors vary from plant to plant. A plant may serve as an excluder for one type of NP and an accumulator for another type (Khan et al., 2019).
Mechanism of NBF mediated plant growth promotion
The synergism of the NP and biofertilizers for promoting plant growth serves as the foundation for the mechanism of NBF-mediated plant growth promotion. NPF promotes plant growth through a wide array of mechanisms, such as (i) increasing nutrient availability and nutrient uptake efficacy of plants, (ii) synthesis of phytohormones (i.e., auxins, gibberellins, cytokinins and abscisic acid), (iii) biocontrol of phytopathogens, (iv) alleviation of biotic and abiotic stresses (such as salinity, drought, flooding, etc.), (v) increasing photosynthate deposition and nutrient transport, and (vi) enhancing photosynthetic activity of plants (Mahapatra et al., 2022; Sharma et al., 2023). The synthesis, characteristics, application methods, and effects of NBF are shown in Figure 1.
Plant growth promoting microorganisms (PGPM) based NBF has been reported to increase the plant growth and yield by increasing (a) nutrient availability (e.g., N2-fixing microorganisms provide N and phosphorus solubilizing microorganisms provide P to the plant) to the plant, (b) photosynthate deposition and nutrient transport, and (c) phytohormone production. Compared to the crop response reported when NPs were added directly as fertilizer, NBF synthesized by enclosing biofertilizers (such as Pseudomonas fluorescens, Bacillus subtilis, and Paenibacillus elgii) in silver or gold NPs was extremely effective in encouraging plant growth in several different agricultural crops (Sharma et al., 2023). Neem cake with PGPR, a nano-scale fertilizer, can increase agricultural yield in leguminous crops by promoting seedling germination and efficiently delivering nutrients to plants (Mala et al., 2017). Chitosan is a high carbon and nitrogen polymer used for the synthesis of nanocompounds that stimulates and supports the growth of beneficial microbes in NBF. Its application increased inorganic nutrient uptake, net photosynthesis ability via enhanced chlorophyll contents, germination rate, shoots height, and root length of tomato plants (Rendina et al., 2019). NBF containing silicon NP aids in improved photosynthesis of plants by enlarging chloroplasts and increasing grana quantity in leaves. It is also synergistically supported by the angle of leaf opening, leaf uprightness, and reduced self-shading, as seen in rice, wheat, and sugarcane (Frew et al., 2018).
Both biotic and abiotic plant stress have harmful consequences on plants, including disruption of the electron transport chain in mitochondria, a reduction in chlorophyll content in chloroplasts, and DNA damage from ROS. NBF reduces plant stress by upregulating genes responsible for the production of antioxidants, osmolytes and stress-related proteins, reducing the harmful effects of ROS on plants and maintaining cell structure and function. An NBF-induced increase in plant resilience by stimulation of pathogenesis-associated proteins (e.g., catalase, peroxidase, polyphenol oxidase, glucanase, chitinase), and nonenzymatic antioxidants (e.g., phenols and flavonoids), has been reported (Dong et al., 2022). Chitosan immobilized silica nanocomposites and chitosan were used to make a NBF containing Glomus mossease, Trichoderma viridae and Bacillus subtilis that could inhibit tomato bacterial wilt caused by Ralstonia solanacearum (Rendina et al., 2019).
Constraints and future perspective
Nano-biofertilizers may have adverse ecological and/or toxicological effects. Crop growth and development may be adversely affected by non-targeted cell death or apoptosis caused by ROS generation and NBFs' unfavorable involvement in cellular metabolism (Wu et al., 2022). These consequences for crops are likely if we are ignorant about the dosage response and mortality of NPs in biological systems. Toxic effects of NBFs can be minimized by using dose metrics that measure particle charge, concentration (number or mass), surface area, and net size as benchmarks for quantifying NP toxicity (Mahapatra et al., 2022). Some NPs used in the formulation of NBF may adversely affect beneficial plant-associated microbes, thereby affecting plant growth and yield. For example, AgNPs have been shown to have a potential antibacterial effect on soil microbial growth at concentrations lower than other heavy metals. Studies have shown that AgNPs can have toxic effects on beneficial microbial communities, including nitrogen-fixing bacteria, ammonifying bacteria, and chemolithotrophic bacteria, negatively affecting plant growth and yield (Bahrulolum et al., 2021). Therefore, it is necessary to select the compatible NPs and biofertilizers and combine NPs and biofertilizers appropriately to produce an efficient, eco- friendly NBF. Although post-nano-based biofertilizer formulations can be used to study ecotoxicological aspects and threats to safety and sanitation for various NBFs, including organic and green NBFs, there is still a high expectation for these nanocommodities to be used throughout the entire agricultural supply chain, including processing, packaging, preservation, transportation, storage, and final delivery.
By offering useful and innovative solutions, nanotechnology is actively participating in tackling a variety of environmental issues. While the field of biomedical sciences has lately improved diagnostic and therapeutic techniques due to phenomenal advancements in nanotechnology, knowledge of the interactions between plants and NBFs is still in its infancy stage. The formulation and application of eco-friendly NBF has received little research so far. Future studies on the parameters below may enable NBF to serve agriculture and transform into a cutting-edge tool for the advancement of sustainable agricultural development.
CONCLUSIONS
With improved overall plant growth dynamics, targeted nutrient delivery, soil fertility, and induced systemic resistance than either NPs or biofertilizers alone, NBFs can pave a new path in crop sustainability. Agriculture would benefit greatly from the use of NBFs, so they must be produced on an industrial scale with stable formulations and environmentally friendly processes. A great deal of effort is being put into utilizing natural resources and implementing biological synthesis methods with several proven advantages, including being environmentally friendly, easy to scale up, and cost-effective; this makes the green synthesis of NBFs an exciting endeavor. There is still a lack of knowledge about the underlying mechanisms that govern the synthesis, action, and stabilization of green synthesized NBFs. To meet the need for NBFs in agriculture, a deeper investigation of biological nanofactories is prompted by the abundance of microorganisms and plants that have been effectively exploited for the synthesis of green NBFs. Research on the bioavailability and biocompatibility of NBF is still in its early phases, and much more needs to be done in this area. A prosperous agro-economy can be established based on NBFs because they can provide favorable conditions for enhanced productivity, improved resource conservation, and a healthier environment under climate change.
REFERENCES
Adrees, M., Khan, Z.S., Ali, S., Hafeez, M., Khalid, S., Rehman, M.Z.U., Hussain, A., Hussain, K., Chatha, S.A.S. and Rizwan, M., 2020. Simultaneous mitigation of cadmium and drought stress in wheat by soil application of iron nanoparticles. Chemosphere 238: 124681.
Akhtar, N. and Ilyas, N., 2022. Role of nanosilicab to boost the activities of metabolites in Triticum aestivum facing drought stress. Plant Soil 1–17.
Akhtar, N., Ilyas, N., Meraj, T.A., Pour-Aboughadareh, A., Sayyed, R.Z., Mashwani, Z.U.R. and Poczai, P., 2022. Improvement of plant responses by Nano-bio-fertilizer: a step towards sustainable agriculture. Nanomaterials 12 (6): 965.
Bahrulolum, H., Nooraei, S., Javanshir, N., Tarrahimofrad, H., Mirbagheri, V.S., Easton, A.J. and Ahmadian, G., 2021. Green synthesis of metal nanoparticles using microorganisms and their application in the agrifood sector. J. Nanobiotechnol. 19(1): 1-26.
Bairwa, P., Kumar, N., Devra, V. and Abd-Elsalam, K.A., 2023. Nano-Biofertilizers Synthesis and Applications in Agroecosystems. Agrochemicals, 2(1): 118-134.
Baragaño, D., Forján, R., Fernández, B., Ayala, J., Afif, E. and Gallego, J.L.R., 2020. Application of biochar, compost and ZVI nanoparticles for the remediation of As, Cu, Pb and Zn polluted soil. Environ. Sci. Pollut. Res. 27: 33681–33691.
Bashir, A., Rizwan, M., Ali, S., Adrees, M. and Qayyum, M.F., 2020. Effect of composted organic amendments and zinc oxide nanoparticles on growth and cadmium accumulation by wheat; a life cycle study. Environ. Sci. Pollut. Res. 27 (19): 23926–23936.
Chen, D., Wang, S., Yin, L. and Deng, X., 2018. How does silicon mediate plant water uptake and loss under water deficiency? Front. Plant Sci. 9: 281.
Daryabeigi Zand, A., Tabrizi, A.M. and Heir, A.V., 2020. The influence of association of plant growth promoting rhizobacteria and zero-valent iron nanoparticles on removal of antimony from soil by Trifolium repens. Environ. Sci. Pollut. Res. 27 (34): 42815–42829.
Das, S., Galgo, S.J., Alam, M.A., Lee, J.G., Hwang, H.Y., Lee, C.H. and Kim, P.J., 2020. Recycling of ferrous slag in agriculture: Potentials and challenges. Cri. Rev. Environ. Sci. Technol. 52(8): pp.1247-1281.
Das, S., Kim, G.W., Lee, J.G., Bhuiyan, M.S.I. and Kim, P.J., 2021. Silicate fertilization improves microbial functional potentials for stress tolerance in arsenic-enriched rice cropping systems. J. Hazard. Mater. 417: 125953.
Davod, T., Reza, Z., Ali, V.A. and Mehrdad, C., 2011. Effects of nanosilver and nitroxin biofertilizer on yield and yield components of potato minitubers. Int. J. Agric. Biol. 13 (6): 986–990.
Dawadi, S., Katuwal, S., Gupta, A., Lamichhane, U., Thapa, R., Jaisi, S., and Parajuli, N., 2021. Current research on silver nano-particles: synthesis, characterization and applications. J. Nanomater. 2021.
de França Bettencourt, G.M., Degenhardt, J., Torres, L.A.Z., de Andrade Tanobe, V.O. and Soccol, C.R., 2020. Green biosynthesis of single and bimetallic nanoparticles of iron and manganese using bacterial auxin complex to act as plant bio-fertilizer. Biocatal. Agric. Biotechnol. 30: 101822.
Detmann, K.C., Araujo, W.L., Martins, S.C., Sanglard, L.M., Reis, J.V., Detmann, E., Rodrigues, F.A., Nunes-Nesi, A., Fernie, A.R. and DaMatta, F.M., 2012. Silicon nutrition increases grain yield, which, in turn, exerts a feed-forward stimulation of photosynthetic rates via enhanced mesophyll conductance and alters primary metabolism in rice. New Phytol. 196 (3): 752–762.
Dong, B.R., Jiang, R., Chen, J.F., Xiao, Y., Lv, Z.Y. and Chen, W.S., 2022. Strategic nanoparticle mediated plant disease resistance. Crit. Rev. Biotechnol. 1–16.
El-Desouky, H.S., Islam, K.R., Bergefurd, B., Gao, G., Harker, T., Abd-El-Dayem, H. and Zewail, R.M., 2021. Nano iron fertilization significantly increases tomato yield by increasing plants'vegetable growth and photosynthetic efficiency. J. Plant Nutr. 44 (11): 1649–1663.
Fatima, F., Pathak, N., Verma, S.R. and Bajpai, P., 2018. Toxicity and immunomodulatory efficacy of biosynthesized silver myconanosomes on pathogenic microbes and macrophage cells. Artif. Cells Nanomed. Biotechnol. 46 (8): 1637–1645.
Frew, A., Weston, L.A., Reynolds, O.L. and Gurr, G.M., 2018. The role of silicon in plant biology: a paradigm shift in research approach. Ann. Bot. 121(7): 1265-1273.
Ghooshchi, F., 2017. Influence of titanium and bio-fertilizers on some agronomic and physiological attributes of triticale exposed to cadmium stress. Glob. NestJ. 19 (3): 458–463.
Hassan, S.E.D., Salem, S.S., Fouda, A., Awad, M.A., El-Gamal, M.S. and Abdo, A.M., 2018. New approach for antimicrobial activity and bio-control of various pathogens by biosynthesized copper nanoparticles using endophytic actinomycetes. J. Radiat. Res. Appl. Sci. 11 (3): 262–270.
Hatami, M., Khanizadeh, P., Bovand, F. and Aghaee, A., 2021. Silicon nanoparticle mediated seed priming and Pseudomonas spp. inoculation augment growth, physiology and antioxidant metabolic status in Melissa officinalis L. plants. Ind. Crops Prod. 162: 113238.
Hernandez-Diaz, J.A., Garza-Garcia, J.J., Zamudio-Ojeda, A., Leon-Morales, J.M., Lopez-Velazquez, J.C. and Garcia-Morales, S., 2021. Plant mediated synthesis of nanoparticles and their antimicrobial activity against phytopathogens. J. Sci. Food Agric. 101 (4): 1270–1287.
Jamdagni, P., Rana, J.S. and Khatri, P., 2018. Comparative study of antifungal effect of green and chemically synthesised silver nanoparticles in combination with carbendazim, mancozeb, and thiram. IET Nanobiotechnol. 12 (8): 1102–1107
Jamkhande, P.G., Ghule, N.W., Bamer, A.H. and Kalaskar, M.G., 2019. Metal nano-particles synthesis: an overview on methods of preparation, advantages and disadvantages and applications. J. Drug Deliv. Sci. Technol. 53: 101174.
Kah, M., Kookana, R.S., Gogos, A. and Bucheli, T.D., 2018. A critical evaluation of nanopesticides and nanofertilizers against their conventional analogues. Nat. Nanotechnol. 13(8): 677-684.
Kah, M., Tufenkji, N. and White, J.C., 2019. Nano-enabled strategies to enhance crop nutrition and protection. Nat. Nanotechnol. 14(6): 532-540.
Kaur, H., Kaur, H., Kaur, H. and Srivastava, S., 2022. The beneficial roles of trace and ultratrace elements in plants. Plant Growth Regul. 1–18.
Khan, M.R., Adam, V., Rizvi, T.F., Zhang, B., Ahamad, F., Jośko, I., Zhu, Y., Yang, M. and Mao, C., 2019. Nanoparticle–plant interactions: two‐way traffic. Small, 15(37): 1901794.
Kumar, S., Sindhu, S.S. and Kumar, R., 2022. Biofertilizers: An ecofriendly technology for nutrient recycling and environmental sustainability. Curr. Res. Microb. Sci. 3: 100094.
Mahapatra, D.M., Satapathy, K.C. and Panda, B., 2022. Biofertilizers and nano-fertilizers for sustainable agriculture: phycoprospects and challenges. Sci. Total Environ. 803: 149990.
Mala, R., Celsia Arul Selvaraj, R., Barathi Sundaram, V., Blessina Siva Shanmuga Rajan, R. and Maheswari Gurusamy, U., 2017. Evaluation of nano structured slow release fertilizer on the soil fertility, yield and nutritional profile of Vigna radiata. Rec. Pat. Nanotechnol. 11 (1): 50–62.
Morsy, N.M., Shams, A.S. and Abdel-Salam, M.A., 2017. Zinc foliar spray on snap beans using nano-Zn with N-soil application using mineral, organic and biofertilizer. Middle East J. Agric. Res. 6 (4): 1301–1312.
Nile, S.H., Thiruvengadam, M., Wang, Y., Samynathan, R., Shariati, M.A., Rebezov, M. and Kai, G., 2022. Nano-priming as emerging seed priming technology for sustainable agriculture recent developments and future perspectives. J. Nanobiotechnol. 20 (1): 1–31.
Ponmurugan, P., Manjukarunambika, K., Elango, V. and Gnanamangai, B.M., 2016. Antifungal activity of bio-synthesised copper nanoparticles evaluated against red root rot disease in tea plants. J. Exp. Nanosci. 11 (13): 1019–1031.
Rehman, M., Liu, L., Wang, Q., Saleem, M.H., Bashir, S., Ullah, S. and Peng, D., 2019. Copper environmental toxicology, recent advances and future outlook: a review. Environ. Sci. Pollut. Res. 26 (18): 18003–18016.
Rendina, N., Nuzzaci, M., Scopa, A., Cuypers, A. and Sofo, A., 2019. Chitosan elicited defense responses in cucumbermosaic virus (CMV) infected tomato plants. J. Plant Physiol. 234: 9–17.
Sharma, B., Tiwari, S., Kumawat, K.C. and Cardinale, M., 2023. Nano-biofertilizers as bio-emerging strategies for sustainable agriculture development: Potentiality and their limitations. Sci. Tot.Environ. 860: 160476.
Singh, P., Kim, Y.J., Zhang, D. and Yang, D.C., 2016. Biological synthesis of nanoparticles from plants and microorganisms. Trends Biotechnol. 34(7): 588-599.
Singh, R.P., Handa, R., and Manchanda, G., 2021. Nanoparticles in sustainable agriculture: an emerging opportunity. J. Control. Release 329: 1234–1248.
Sonawane, H., Arya, S., Math, S. and Shelke, D., 2021. Myco-synthesized silver and titanium oxide nanoparticles as seed priming agents to promote seed germination and seedling growth of Solanum lycopersicum: a comparative study. Int. Nano Lett. 11 (4): 371–379.
Sonawane, H., Shelke, D., Chambhare, M., Dixit, N., Math, S., Sen, S., Borah, S.N., Islam, N.F., Joshi, S.J., Yousaf, B. and Rinklebe, J., 2022. Fungi-derived agriculturally important nanoparticles and their application in crop stress management–Prospects and environmental risks. Environ. Res. 212: 113543.
Tan, W., Peralta-Videa, J.R. and Gardea-Torresdey, J.L., 2018. Interaction of titanium dioxide nanoparticles with soil components and plants: current knowledge and future research needs: a critical review. Environ. Sci. Nano 5 (2): 257–278.
Tiwari, M., Jain, P., Hariharapura, R.C., Narayanan, K., Bhat, U., Udupa, N. and Rao, J.V., 2016. Biosynthesis of copper nanoparticles using copper-resistant Bacillus cereus, a soil isolate. Process Biochem. 51 (10): 1348–1356.
Wu, H. and Li, Z., 2022. Nano-enabled agriculture: how nanoparticles cross barriers in plants?. Plant Communications.
Yasmeen, F., Razzaq, A., Iqbal, M.N. and Jhanzab, H.M., 2015. Effect of silver, copper and iron nanoparticles on wheat germination. Int. J. Biosci. 6: 112–117.