DOI: https://doi.org/10.56669/HYZL2696
ABSTRACT
The growing concerns among consumers and governments regarding the issues associated with synthetic chemicals in disease control and food safety have prompted growers to seek eco-friendly alternatives to traditional chemical-based practices. In response to increasing environmental awareness, the use of biocontrol agents for plant disease suppression is emerging as a viable alternative. Biological control relies on the complex interactions (agonistic and antagonistic)—between plants and microbes living in the rhizosphere and phyllosphere. Various organisms including fungi, bacteria, mycorrhiza, yeast, virus, algae, cyanobacteria and so on, were identified with biological control properties and thus can be applied in plant disease management. Research on the selection, evaluation, characterization and application of these agents has significantly increased over the past few decades. However, despite extensive global research, the adoption of biopesticides by farmers remains limited. To promote the development and effective use of bioformulations, it is essential to optimize the activity of biocontrol agents, share knowledge on formulation processes, and simplify registration procedures. This paper discusses the advancements, challenges and perspectives related to the application of novel biocontrol agents in plant disease management.
Keywords: Biocontrol, Modes of action, Research and development, Control
INTRODUCTION
Plant diseases caused by infectious pathogens have significantly impacted human society and the natural environment, damaging food production, economic development, ecological resilience, and landscapes throughout human history. Plant diseases result from complex interactions among plants, pathogens, and the environment. Plant diseases can occur in the entire crop production chain and remain as one of the greatest threats to the sustainable development of society (Savary et al., 2019). According to the Food and Agriculture Organization (FAO), 14% of global crop production losses are caused by plant diseases each year, with fungal diseases accounting for 42% and bacteria for 27% (Roca-Couso et al., 2021). In the long history of agriculture, humans have developed a variety of approaches to manipulate the interaction to create a system in favor of the growth and development of host plants but suboptimum to the establishment, reproduction, and transmission of pathogens (Bisht et al., 2020). Depending on circumstances of crop, pathogen, geographic location, technology availability, regulation policy, and other factors, these control approaches can be agronomic (viz., crop diversification and field hygiene), regulative (viz., quarantine and eradication), genetic (viz., disease resistance and tolerance), physical (viz., soil solarization and flooding), chemical (viz., pesticides and host-immunity inducer) and can be used individually or in combination (integrated disease management, IDM) to suppress causal pathogen, promote host immunity, or change the biotic and abiotic environment where host–pathogen interaction occurs (He et al., 2021). Over the past 50 years, the most common method for disease control has been the extensive use of chemical pesticides (Peshin et al., 2009; Peshin and Zhang, 2014; Zhang et al., 2011; Zhang, 2018). Chemical and synthetic pesticides are used in excessive amounts to control pests in crop fields and such excessive applications deteriorate soil fertility and ecosystem health (Zhang et al., 2011; Zhang, 2018). Concerns about the safety of chemical residues and possible emergence of pesticide resistance in some pest species have necessitated the development of substitutes for these synthetic agro-inputs. However, the high effectiveness and ease of utilization of these chemicals resulted in environmental contamination and the presence of pesticide residues in food, in addition to various social and economic problems. Today, in the light of growing concerns towards environmental safety, suppression of plant diseases through biocontrol agents (BCAs) is gaining ground as an alternative to traditional disease management strategies. Now it is essential to focus on the challenges involved in testing, formulating, and delivering newer potential biocontrol agents within the context of integrated disease management. The objective of this paper is to build-on recent detailed reviews on advances, challenges and perspectives in the application of novel biocontrol agents for plant disease management.
BIOCONTROL AGENTS
Organisms that originate from nature and are utilized to control pests, weeds and diseases are known as biocontrol agents. Biocontrol agents can be classified into four distinct types: i) Macrobials, which encompass insects, mites, and beneficial nematodes that target pests; ii) Microbials, which are microorganisms like bacteria, fungi, and viruses, along with their derivatives. Microbial products may include these microorganisms, their metabolites, or fragments of their cells, and they can combat pests through direct infection, competitive displacement, or by forming a physical barrier; iii) Semiochemicals, chemical compounds emitted by plants and animals that send signals capable of influencing pest behavior. These are employed as repellents, attractants, or to disrupt mating; iv) Natural substances sourced from plants, minerals, or animals, or artificially created to replicate these natural compounds. They serve to repel and control both microbes and insects (Kumar et al., 2019). Additionally, it encompasses modified organisms, genes or a gene product used to suppress the growth of the unwanted organism (pest/pathogen) and to enhance the growth and yield of the wanted organism (crops, microorganism, or insects). The use of biocontrol agents to control plant diseases and pests is called biocontrol. It is a method of plant disease management by inhibiting plant pathogens, improving plant immunity, and/or modifying the environment through the effects of beneficial microorganisms, compounds, or healthy cropping systems (Burketova et al., 2015). Biological control offers several advantages over other approaches of plant disease management (Figure 1).
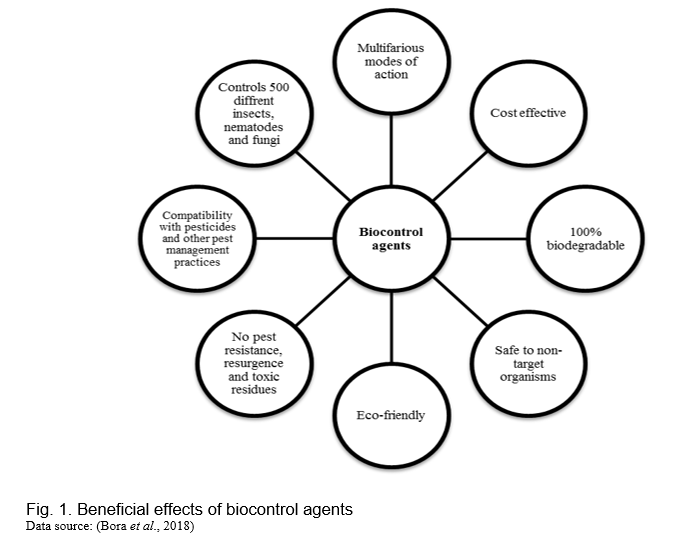
MECHANISMS EMPLOYED BY BIOCONTROL AGENTS FOR MANAGEMENT OF PLANT DISEASES
Biological control agents protect crops from diseases caused by plant pathogens via different modes of action. The biocontrol activity is exerted either directly through antagonism of plant pathogens or indirectly by eliciting a plant-mediated resistance response. Direct antagonism results from physical contact and/or a high degree of selectivity for the pathogen, by different mechanism(s) expressed by the biocontrol agents. Indirect antagonism results from activities that do not involve sensing or targeting a pathogen by the biocontrol agent/s (Figure 2). Stimulation of plant host defence pathways by biocontrol agents is the most indirect form of antagonism (Köhl et al., 2019; Hegde et al., 2022).
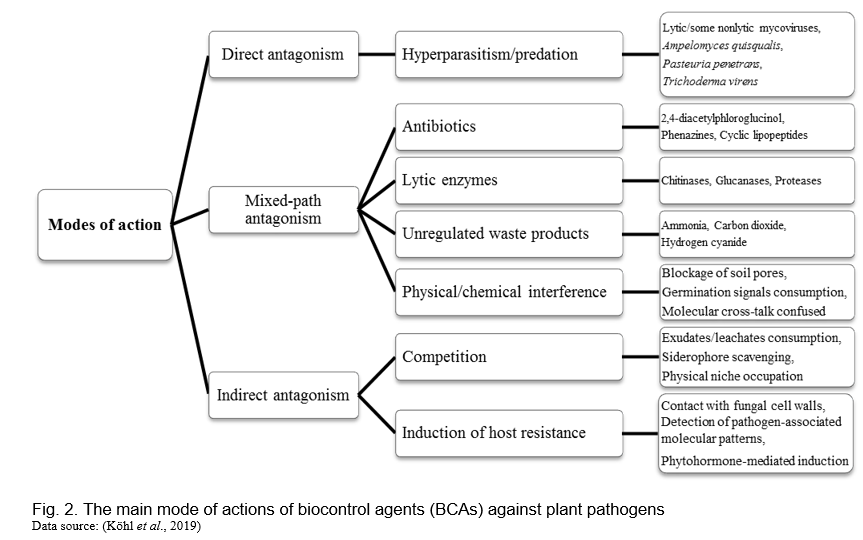
Biological control is dependent on numerous agonistic and antagonistic interconnections between plants and microbes living in the rhizosphere and phyllosphere (Mishra et al., 2015) and their application to minimize disease and subdue pests. Organisms from the rhizosphere can be harnessed from the surrounding environment (the black box approach) or can be introduced into the field from external sources (the silver bullet approach). It is beneficial to apply a consortium of microbes with collaborative properties rather than relying on a single organism since microbial consortia make up a stable rhizosphere that offers more effective control against pathogens (Ram et al., 2018). Apart from microbial applications, the utilization of other plant products like extracts, biofertilizers, and biopesticides, natural enemies of pests and pathogens, and gene products also aid in carrying out biological control (Ab et al., 2018).
ADVANCES IN RESEARCH AND DEVELOPMENT OF NOVEL BIOCONTROL AGENTS IN MANAGEMENT OF PLANT DISEASES
There is an increasing preference for food produced in ways that impart as little environmental impact as possible. Biocontrol agents provide a natural and environmentally acceptable alternative to conventional pesticides, therefore lowering agriculture’s ecological imprint. Biocontrol agents play an important part in sustainable agriculture by promoting natural pest control processes and lowering the need for synthetic pesticides. Government rules and incentives aimed at improving sustainable agriculture increase the market for biocontrol chemicals. Therefore, the transition to more environment-friendly pest management corresponds with the usage of biocontrol agents, boosting market expansion as farmers seek effective and ecologically favorable solutions. Furthermore, customers’ growing desire for organic products fuels this trend, forcing farmers to invest in biocontrol methods to fulfil market needs while remaining organically certified. According to research, global biocontrol agent’s market was valued at US$5.8 billion in 2023 and is expected to reach US$10.6 billion by 2032, at a CAGR of 8.1% during the forecast period. Based on formulation, the liquid formulation segment dominated the market in 2022 with a market share of 40% and is expected to keep its dominance during the 2024-2032 forecast period. Based on the application method, the foliar spray segment dominated the market in 2022 with a market share of 55% and is expected to keep its dominance during the 2024-2032 forecast period. By region, Biocontrol agent’s market is segmented into North America, Europe, Asia-Pacific, Latin America, the Middle East & Africa. The Asia-Pacific dominated the global biocontrol agents market in 2022 with a market share of 45% and is expected to keep its dominance during the forecast 2024-2032 period (www.custommarketinsight.com). Overall, biocontrol agent’s registrations are increasing globally, the expansion of various technologies has increased the scope for more products and the change in the trend to develop biocontrol agents is definitely on the rise (Figure 3).
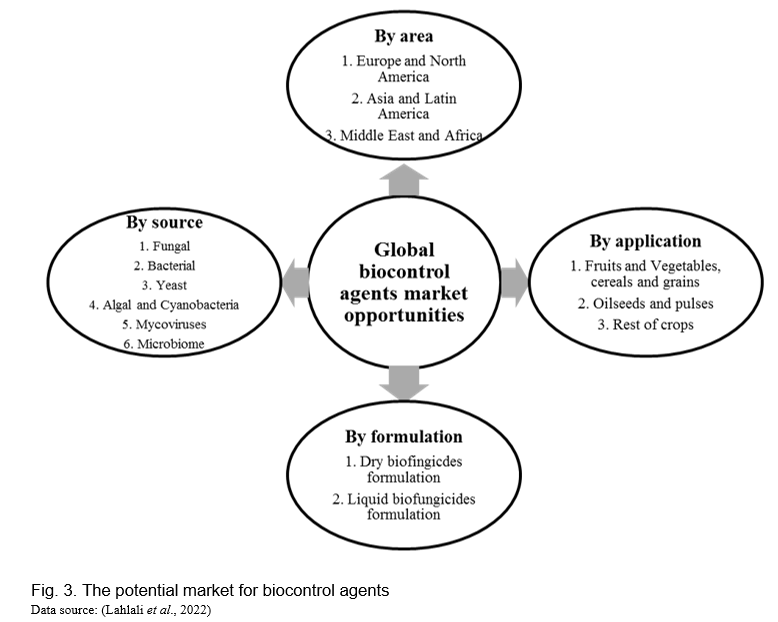
A group of novel biocontrol agents that effectively control diseases in economically important crop species are discussed (Table 1).
Table 1. Groups of successful biocontrol agents that effectively control diseases in economically important crop species
Groups
|
Biocontrol agent
|
Target pathogens
|
Crops
|
Diseases
|
References
|
Fungi
|
|
|
|
|
|
|
T. harzianum
|
Crinipellis perniciosa
|
Cocoa
|
Witches’ broom
|
Marco et al. (2003)
|
|
T. lignorum
|
R. solani
|
Bean
|
Damping off
|
Aziz et al. (1997)
|
|
T. viride,
T. pseudokoningii,
T. koningii
|
Sclerotium cepivorum
|
Onion
|
White rot
|
Clarkson et al. (2002)
|
|
T. harzianum
|
Penicillium expansum
|
Apple
|
Blue and grey mould
|
Batta (2004)
|
|
T. virens, T. harzianum
|
R. solani
|
Potato
|
Stem canker or black scurf
|
Brewer and Larkin (2005)
|
|
T. koningii, T. aureoviride,
T. longibrachiatum
|
Sclerotinia sclerotiorum
|
Sunflower
|
Head rot
|
Escande et al. (2002)
|
|
T. asperellum
|
F. oxysporum
|
Tomato
|
Wilt
|
Cotxarrera et al. (2002)
|
|
T. koningii
|
Gaeumannomyces graminis var. tritici
|
Wheat
|
Take-all disease
|
Simon (1989)
|
|
T. virens
|
Verticillium dahliae
|
Cotton
|
Wilt
|
Hanson (2000)
|
|
T. afroharzianum
|
Uncinula necator
|
Grapes
|
Powdery mildew
|
Saxena et al. (2021)
|
|
Purpureocillium lilacinum (=Paecilomyces lilacinus) IIHR- Pl-2 (NAIMCC-SF-0034/ITCC 6887)
|
Meloidogyne incognita
|
Brinjal, tomato, carrot and okra
|
Root knot nematode
|
Saxena et al. (2021)
|
|
Pochonia chlamydosporia (=Verticillium chlamydosporium) IIHR- Vc-3(NAIMCC-SF-0035/ITCC 6898)
|
Meloidogyne incognita
|
Brinjal, tomato, carrot and okra
|
Root knot nematode
|
Saxena et al. (2021)
|
|
Trichoderma viride + Trichoderma harzianum
|
Pythium, Rhizoctonia,
Phytophthora and Fusarium
|
Many hosts
|
–
|
Saxena et al. (2021)
|
|
Pochonia chlamydosporia
|
Fusarium oxysporum f.sp. ciceris and F. udum, Meloidogyne incognita
|
Chickpea and redgram
|
Wilt disease complex
|
Saxena et al. (2021)
|
|
Gliocladium. spp.
|
Fusarium, Rhizoctonia,
Sclerotium, and Pythium
|
Many hosts
|
Soil borne diseases
|
Saxena et al. (2021)
|
|
Trichoderma reesei CSR-T-3 (NAIMCC-SF-0030)
|
Fusarium oxysporum f. sp. cubense Tropical race 4 and race 1
|
Banana
|
Fusarium wilt
|
Saxena et al. (2021)
|
|
Aspergillus niger AN-27
|
Pythium, Fusarium,
Macrophomina,
Rhizoctonia and Sclerotinia
|
–
|
–
|
Prashant et al. (2016)
|
|
Trichoderma asperelloides 5R (NAIMCC-SF-0026)
|
Uncinula necator
|
Grapes
|
Powdery mildew
|
Saxena et al. (2021)
|
|
Trichoderma harzianum IARI P-4(MTCC 5371)
|
Fusarium oxysporum f. sp. ciceris, Sclerotium rolfsii, Sclerotinia sclerotiorum,
Rhizoctonia solani, R. bataticola, Fusarium
oxysporum f. sp. lycopersici, Pythium ultimum
|
Chickpea, tomato, mungbean French bean
|
Soil borne diseases
|
Saxena et al. (2021)
|
|
Trichoderma viride
|
Fusarium, R. solani,
Macrophomina phaseolina and Colletotrichum
|
Many hosts
|
Soil borne diseases
|
Prashant et al. (2016)
|
|
Trichoderma harzianum Th4d (NAIMCC-F-02188)
|
Phytophthora sp., Macrophomina phaseolina, Fusarium sp., Botrytis cinerea, Alternaria sp. and Golovinomyces cichoracearum
|
Safflower, castor, aster, sunflower
|
Seedling blight, root rot, wilt, grey mold, leaf blight, powdery mildew
|
Saxena et al. (2021)
|
|
Trichoderma erinaceum
|
Rhizoctonia solani, Sclerotium rolfsii, Helminthosporium oryzae and Sclerotium oryzae
|
Rice
|
Soil borne plant pathogens, brown leaf spot
|
Swain et al. (2018)
|
|
Bjerkandera adusta BK-1
|
–
|
Tomato
|
Grey mould, brown spot, and early blight
|
Chen et al. (2021)
|
Yeast groups
|
|
|
|
|
|
|
Cryptococcus albidus var. aerius, Pichia guilliermondii,
Debaryomyces hansenii
|
Botryodiplodia theobromae
|
Mango
|
Post-harvest rot
|
Sugiprihatini et al. (2011)
|
|
P. guilliermondii
|
Aspergillus flavus
|
Soybean
|
Post-harvest infection
|
Wisniewski et al. (1991)
|
|
P. membranefaciens, C. albidus
|
Monilinia fructicola, Penicillium
expansum, Rhizopus
stolonifer
|
Apple
|
Post-harvest
infection
|
Chan and Tian
(2005)
|
|
P. guilliermondii
|
P. digitatum
|
Citrus
|
Decay of citrus fruit
|
Droby et al. (1993)
|
|
C. laurentii
|
M. fructicola, P. expansum
|
Peach
|
–
|
Yao and Tian (2005)
|
|
Chaetomium globosum
|
Phoma betae, P. ultimum, R. solani
|
Sugar beet
|
Damping-off
|
Walther and
Gindrat (1988)
|
Oomycetes
|
|
|
|
|
|
|
Pythium oligandrum
|
Sclerotinia sclerotiorum
|
Wheat
|
White mould/stem rot
|
Madsen and de
Neergaard (1999)
|
|
|
Verticillium dahliae
|
Pepper
|
Wilt
|
Rekanovic et al. (2007)
|
|
|
Pythium dissotocum
|
Tomato
|
Root rot
|
Vallance et al. (2009)
|
|
|
P. parasitica
|
Tomato
|
Buckeye rot
|
Picard et al. (2000)
|
|
|
B. cinerea
|
Tomato
|
Grey mould
|
Lou et al. (2011)
|
|
|
R. solani
|
Potato
|
Black scurf
|
Ikeda et al. (2012)
|
|
|
Aphanomyces cochlioides
|
Sugar beet
|
Root rot
|
Takenaka and
Ishikawa (2013)
|
|
|
Cercospora beticola
|
Sugar beet
|
Leaf spot
|
Takenaka and
Tamagake (2009)
|
|
|
Phytoplasma
|
Tobacco
|
–
|
Lherminier et al. (2003)
|
Mycorrhiza
|
|
|
|
|
|
|
Glomus mosseae
|
Thielaviopsis basicola
|
Tobacco
|
Root infection
|
Baltruschat and
Schoenbeck (1975)
|
|
Vesicular arbuscular mycorrhiza
|
R. solani
|
Rape seed
|
Damping-off
|
Iqbal et al. (1977)
|
|
Glomus fasciculatus
|
S. rolfsii
|
Peanut
|
Southern blight
|
Krishna and
Bagyaraj (1983)
|
Bacteria
|
|
|
|
|
|
|
Pseudomonas chlororaphis
|
F. oxysporum f. sp. radicislycopersici
|
Tomato
|
Foot and root
rot
|
Bolwerk et al. (2003)
|
|
P. fluorescens
|
Phytophthora infestans
|
Potato
|
Late blight
|
Hunziker et al. (2015)
|
|
|
Sarocladium oryzae
|
Rice
|
Sheath rot
|
Saravanakumar et al. (2009)
|
|
|
Banana bunchy top virus
|
Banana
|
Bunchy top disease
|
Kavino et al. (2008)
|
|
|
Dematophora necatrix
|
Avocado
|
Root rot
|
Cazorla et al. (2006)
|
|
Pseudomonas fluorescens IIHR Pf-2 (NAIMCC-SB-0038/ITCC B0034) and Trichoderma harzianum IIHR Th-2 (NAIMCC-SF-0033/ITCC 6888)
|
Meloidogyne incognita, Ralstonia solanacearum, Erwinia carotovora, Fusarium oxysporum f. sp. vasinfectum, Fusarium solani
|
Tomato, cotton
|
Root knot nematode, bacterial wilt, vascular wilts
|
Saxena et al. (2021)
|
|
Pseudomonas fluorescens
(NAIMCC-SB-0053)
|
–
|
Wheat, rice tomato, chickpea
|
Spot blotch, sheath blight and wilt
|
Saxena et al. (2021)
|
|
P. putida
|
Fusarium sp.
|
Radish
|
Wilt
|
de Boer et al. (2003)
|
|
Bacillus pumilus, B. amyloliquefaciens, B. subtilis
|
Cucumber mosaic virus
|
Tomato
|
Mosaic disease
|
Zehnder et al. (2000)
|
|
B. amyloliquefaciens, B. subtilis, B. pumilus
|
Tomato mottle virus
|
Tomato
|
Tomato mottle
|
Murphy et al. (2000)
|
|
B. pumilus
|
Erwinia tracheiphila
|
Cucumber
|
Bacterial wilt
|
Zehnder et al. (2001)
|
|
|
Peronospora tabacina
|
Tobacco
|
Blue mould
|
Zhang et al. (2002)
|
|
B. subtilis, B. pumilus
|
Sclerospora graminicola
|
Pearl millet
|
Downy mildew
|
Niranjan Raj et al. (2003)
|
|
B. cereus
|
Corynespora cassiicola
|
Tomato
|
Foliar diseases
|
Silva et al. (2004)
|
|
Bacillus sp.
|
Phytophthora capsici
|
Bell pepper
|
Blight
|
Jiang et al. (2006)
|
|
|
Magnaporthe grisea
|
Rice
|
Blast
|
Naureen et al. (2009)
|
|
|
P. capsici
|
Squash
|
Blight
|
Zhang et al. (2010)
|
|
B. amyloliquefaciens
|
F. oxysporum
|
Maize
|
Wilt
|
Koumoutsi et al. (2004)
|
|
Brevibacillus brevis
|
B. cinerea
|
Cucumber
|
Grey mould
|
Konstantinidou-Doltsinis et al. (2002)
|
|
Enterobacter agglomerans
|
R. solani
|
Cotton
|
Root rot
|
Chernin et al. (1995, 1997)
|
|
Paenibacillus sp.
|
F. oxysporum
|
Sorghum
|
Wilt
|
Budi et al. (2000)
|
|
Lysobacter enzymogenes
|
F. graminearum
|
Wheat
|
Wilt
|
Li et al. (2008)
|
|
Stenotrophomonas maltophilia
|
P. ultimum
|
Sugar beet
|
Damping-off
|
Dunne et al. (2000)
|
|
Agrobacterium radiobacter
|
Agrobacterium tumefaciens
|
Many hosts
|
–
|
Vicedo et al. (1993)
|
|
Collimonas fungivorans
|
F. oxysporum
|
Tomato
|
Wilt
|
Kamilova et al. (2007)
|
|
L. enzymogenes
|
Pythium aphanidermatum
|
Cucumber
|
Root and crown rot
|
Folman et al. (2003)
|
|
Burkholderia cepacia
|
Pythium sp. and
Aphanomyces sp.
|
Peas
|
Damping off,
root rot
|
Heungens and
Parke (2000)
|
|
Bacillus velezensis K01
|
Botrytis cinerea
|
Tomato and pepper
|
–
|
Xue et al. (2023)
|
Actinobacteria
|
|
|
|
|
|
|
S. janthimts,
S. cinerochromogenes
|
Pythium coloration
|
Carrot
|
Cavity spot
|
El-Tarabily et al. (1997)
|
|
Streptomyces species
|
Phytophthora medicaginis and Phytophthora sojae
|
Alfalfa and soybean
|
Root rot
|
Xiao et al. (2002)
|
|
|
Plectosporium tabacinum
|
Lupin
|
Root rot
|
El-Tarabily (2003)
|
|
|
Pythium aphanidermatum
|
Cucumber
|
Damping-off
|
El-Tarabily (2006)
|
|
Actinomadura sp.
|
Phytophthora cinnamomi
|
Snapdragon
|
Root rot
|
You et al. (1996)
|
|
Micromonospora sp.
|
Gaeumannomyces graminis var. tritici
|
Wheat
|
Take all
|
Coombs et al. (2004)
|
|
|
Fusarium oxysporum f. sp. lycopersici
|
Tomato
|
Wilt
|
Smith (1957)
|
|
M. carbonacea
|
P. coloratum
|
Carrots
|
Cavity spot
|
El-Tarabily et al. (1997)
|
|
|
Sclerotinia minor
|
Lettuce
|
Basal drop
|
El-Tarabily et al. (2000)
|
|
M. chalcea
|
P. aphanidermatum
|
Cucumber
|
Damping-off
|
El-Tarabily (2006)
|
|
Microbispora rosea
|
P. aphanidermatum
|
Cucumber
|
Damping-off
|
El-Tarabily (2006)
|
|
|
G. graminis var. tritici
|
Wheat
|
Take all
|
Coombs et al. (2004)
|
|
Streptosporangium albidum,
Streptoverticillium netropsis
|
P. coloratum
|
Carrots
|
Cavity spot
|
El-Tarabily et al. (1997)
|
|
Arthrobacter sp.
|
Fusarium moniliforme var. subglutinans
|
Pine
|
Pitch canker
|
Barrows-Broaddus and
Kerr (1981)
|
Virus
|
|
|
|
|
|
|
Zucchini yellow mosaic virus (mild strain)
|
Severe strains of same
|
Cucurbits
|
Mosaic
|
Lecoq and Lemaire (1991)
|
|
Papaya ringspot virus (mild strain)
|
Severe strains of same
|
Papaya
|
Ring spot disease
|
Tennant et al. (1994)
|
|
Mild strain of Citrus tristeza virus (mild strain)
|
Severe strains of same
|
Citrus
|
Tristeza
|
Folimonova (2013)
|
Fungal agents for biocontrol
The well-known fungal biocontrol agents include the Trichoderma species, ectomycorrhizas, arbuscular mycorrhizas (AMF), yeasts and endophytes. Even the nonvirulent strains of certain pathogens can utilize hypovirulence-associated mycoviruses in order to function as biocontrol fungi (Ghorbanpour et al., 2018). There are 300 fungal antagonists belonging to 13 classes and 113 genera that were used against fungal plant pathogens. Trichoderma is identified as the genus (comprising 25 species) with greatest potential of biocontrol and is used as a biocontrol agent against a number of plant fungal diseases. Trichoderma species namely T. atroviride, T. hamatum, T. harzianum and T. viride are effective in the control of soil borne fungal pathogens (Hegde and Vijaykumar, 2023). In addition, some more antagonistic species, Alternaria, Aspergillus, Candida, Fusarium, Gliocladium, Penicillium, Pichia, Pythium, Talaromyces and Verticillium play important roles in the management of plant diseases (Hegde and Jahagirdar, 2020; Kasun et al., 2020). Microbial communities can inhabit both external surfaces (epiphytes) or internal plant tissues (endophytes), and these communities play an important role in protecting plants from diseases (Parasuraman et al., 2019). Numerous metabolites with various chemical structures, including terpenoids, alkaloids, steroids, peptides, isocoumarins, benzopyranones, and quinones, have been identified in endophytic fungi. The identification of these metabolites provided a solid chemical basis for the development of agrochemicals that may have antibacterial, antifungal, herbicidal, nematicidal, insecticidal and other agricultural uses (Risoli et al., 2022). Biocontrol agents Chaetomium sp. and Athelia bombacina were successful in suppression of Venturia inaequalis; Rhodotorula kratochvilovae strain LS11 controlled Monilinia spp., while Tuberculina maxima parasitizes the white pine blister rust fungus Cronartium ribicola; Darluca filum and Verticillium lecanii parasitize several rusts, Tilletiopsis sp. parasitizes the cucumber powdery mildew fungus Sphaerotheca fuliginea, and Nectria inventa and Gonatobotrys simplex parasitize two pathogenic species of Alternaria (Agrios, 2005). A novel isolate, Trichoderma erinaceum isolated from above-ground habitats effectively controlled three soil borne plant pathogens of rice i.e., Rhizoctonia solani, Sclerotium rolfsii and Sclerotium oryzae under controlled conditions and R. solani and Helminthosporium oryzae under field conditions while improving the germination rate and vigor of rice plant (Swain et al., 2018). The fungal strain, Bjerkandera adusta BK-1, is a novel promising broad-spectrum biocontrol agent against multiple plant fungal diseases (Chen et al., 2021).
Mycorrhizal fungi as biocontrol agents
Several studies lay emphasis on the biocontrol abilities of Arbuscular mycorrhizal fungi (AMF) as they have been shown to reduce the incidence of fungal diseases and nematode attacks on host plants by 30 to 42% and 44–57%, respectively (Veresoglou and Rillig, 2012; Mishra et al., 2018). AMF offers defence against several fungal pathogens belonging to the genera Colletotrichum, Alternaria, Erysiphe, Gaeumannomyces, Macrophomina, Botrytis, Rhizoctonia, Fusarium, Cylindrocladium, Sclerotium and Verticillium (Singh, 2017). Notable ectomycorrhizal species such as Amanita spp., Boletinus spp., Cenococcum spp., Lactarius spp., Laccaria laccata, Pisolithus tinctorius, Suillus luteus and Thelephora terrestris are known to generate metabolites that safeguard plant root systems from diseases (Trappe, 1962). Suillus luteus has been identified as an effective bio control agent against damping-off disease caused by Fusarium verticillioides and Fusarium oxysporum in Scots and Stone pine trees (Mateos et al. 2017).
Biocontrol by yeasts
Yeasts such as Aureobasidium pullulans, Cryptococcus albidus, Candida oleophila, Saccharomyces cerevisiae, and Metschnikowia fructicola are currently being employed as biocontrol agents as they are effective adversaries of various plant pathogens (Pandit et al. 2022). Biofungicides based on Aureobasidium pullulans strains indicated for biocontrol applications against fungal pathogens of fruit (e.g., Boni protect®) are available in the market (Weiss et al., 2006). The BCA Papiliotrema terrestris strain LS28 was isolated from apple epiphytic microflora and selected for its ability to counteract fungal pathogens of plants and fruits, both in the field and in postharvest stages (Palmieri et al., 2021). Low temperature-adapted yeasts Leucosporidium scottii and Cryptococcus laurentii were highly effective in their biocontrol activity on apple and tomatoes inoculated with Botrytis cinerea in cold storage conditions (Hu et al., 2017).
Bacteria for biocontrol
A wide variety of bacterial genera, including Agrobacterium, Alcaligenes, Arthrobacter, Bacillus, Enterobacter, Erwinia, Pseudomonas, Rhizobium, Serratia, Stenotrophomonas, Streptomyces, Xanthomonas have been described to have plant disease protection activity against fungal and bacterial pathogens (Bonaterra et al., 2022). The activity of P. fluorescens EPS62e and P. pseudoalcaligenes AVO110 in the reduction of Erwinia amylovora or Rosellinia necatrix infections, respectively, is based on their strong fitness in colonizing plant tissues as they have higher growth potential and nutrient use efficiency than the target pathogens (Cabrefiga et al., 2007; Pliego et al., 2008, Dobhal and Hegde, 2021). In Vitis, P. fluorescens PTA-CT2 induces ISR to Plasmopara viticola and Botrytis cinerea that depends on the activation of SA or JA and ABA defensive pathways (Lakkis et al., 2019). Species of Bacillus including B. subtilis, B. amyloliquefaciens, B. methylotrophicus, B. polymyxa, B. cereus, B. coagulans, B. subterraneous, B. licheniformis, B. pumilus, B. circulans, B. velezensis, B. megaterium, B. firmus, B. aquimaris, B. vietnamensis, and B. aerophilus have been reported to be suitable for biological control of plant disease and plant growth promotion (Fan et al., 2017; Saxena et al., 2020; Hegde and Malligawad, 2020). Bacillus spp. produce several bacteriocins with antimicrobial activity such as amylolysin, amylocyclicin, amysin, subtilin, subtilosin A, subtilosin B, thuricin. Bac-GM17 produced by B. clausii GM17 have activity against Agrobacterium tumefaciens (Mouloud et al., 2013) or thuricin Bn1 from B. thuringiensis subsp. kurstaki Bn1 against Pseudomonas savastanoi and Pseudomonas syringae (Ugras et al., 2013). B. amyloliquefaciens FZB42 produced secondary metabolites (surfactin, fengycin, and bacillomycin D) that trigger plant defense gene expression and contribute to lettuce bottom rot reduction (Chowdhury et al., 2015). A novel biocontrol agent Bacillus velezensis K01 has a broad-spectrum antagonistic activity and demonstrated a biocontrol efficiency of over 78% against preharvest and postharvest grey mold diseases in tomato and pepper (Xue et al., 2023). Streptomyces CBQ-EA-2 and CBQ-B-8 have chitinolytic, cellulolytic, and proteolytic activity and result in reduced Macrophomina phaseolina and Rhizoctonia solani infections in Phaseolus vulgaris (Díaz-Díaz et al., 2022). Pantoea species were shown to target a wide spectrum of plant pathogens including bacteria, fungi, and oomycetes via secretion of antimicrobial compounds such as pantocins, herbicolins, microcins, and phenazines (Walterson and Stavrinides, 2015). Lactobacillus plantarum PM411 and TC92 are effective in preventing bacterial plant diseases (Daranas et al., 2019).
Plant viruses and their biocontrol nature
Viruses are not regarded as living organisms, but certainly as valid agents of biological control, and hence they are included (with these caveats) within our definition of living agents (Stenberg et al., 2021). Viruses have been increasingly used to combat pests both directly (by pathogenic pest infection) and indirectly (by cross-protection, i.e., ‘vaccinating’ crops with mild virus strains), and placed in the canon of biological control with no conceptual opposition from the scientific community (Di Giallonardo and Holmes, 2015). Qu et al. (2021) have elucidated the effects of a single-stranded DNA virus, Sclerotinia sclerotiorum hypovirulence-associated DNA virus 1 (SsHADV-1) that infects the fungus Sclerotinia sclerotiorum, a disease causative agent of many crops and they have further elucidated the altered expression of phenotype related genes upon SsHADV-1 infection by using digital RNA sequencing.
Phage-based biocontrol
Phages have been in use as biocontrol agents against bacterial pathogens for a long time. Peptidoglycan hydrolases, lysins from phages Atu_ph02 and Atu_ph03 are capable of blocking cell division in Agrobacterium tumefaciens (causes crown gall disease) resulting in its lysis (Attai et al., 2017). Other lysins from CMP1 and CN77 phages have also shown lytic capacity against Clavibacter michiganensis subsp. michiganensis, that cause bacterial wilt and canker of tomato (Wittmann et al., 2016). Mostly, the application of phages and phage lysins in plant disease management is a progressive step and has shown positive outcomes in a number of instances. Focus now needs to be on developing better delivery methods and guaranteeing a longer shelf life for the phage and its enzymes on the host plant (Dy et al., 2018) to facilitate effective biocontrol.
Algal and cyanobacterial biocontrol activities
Algal and cyanobacterial extracts are a rich source of bioactive elicitors (Jimenez et al., 2011) with antifungal, antiviral and antibacterial properties (Arunkumar et al., 2010). Activity of polyphenol oxidase and peroxidase enzymes improved when extracts from Cystoseira myriophylloides, Laminaria digitata and Fucus spiralis were utilized against Verticillium dahliae wilt of tomato (Esserti et al., 2017). Employing Nostoc entophytum and Nostoc muscorum in the soil against Rhizoctonia solani greatly enhance seedling endurance along with improving root and shoot dry weight and plant length (Osman et al., 2011). Usage of Cyanobacteria, Anabaena sp. on zucchini cotyledons infected with powdery mildew (Podosphaera xanthii) resulted in enhanced enzymatic activity of peroxidases, endochitinase, chitin 1,4-β-chitotriosidase, β-N-acetylhexosaminidase, and β-1,3-glucanase (Roberti et al., 2015).
ADVANCED BIOCONTROL STRATEGIES
FOR THE MANAGEMENT OF PLANT DISEASES
Microbial volatilome and its role in the biological control
Among the several microbiological strategies used by BCAs, the production of volatile organic compounds (VOCs) is a method that is helpful in situations where the straightforward association between the pathogen and its competitor is not possible. All living forms synthesize VOCs, and these can be exploited for usage in biocontrol of plant pathogens like bacteria, oomycetes, and fungi. VOCs are a sustainable preference for synthetic fungicides due to their ease of application, low residue deposition in the environment and on crops, and their biocontrol efficacy (Tilocca et al., 2020). In vitro studies indicated that Bacillus volatile compounds reduced Ralstonia growth and viability and caused significant problems in cell integrity and motility in addition to considerable alterations in Ralstonia genes expression that controls tobacco wilt disease progression (Tahir et al., 2017). It’s possible that bacterial volatiles have a role in Bacillus reported biocontrol properties both directly and indirectly, and that bacterial VOC bouquets function as multifactorial, sequential, or simultaneous signals on pathogens and hosts (Bailly and Weisskopf, 2017).
Microbiome-based solutions for plant protection
A wide range of microorganisms effectively interact with plants in the rhizosphere region. Microbial invasion can affect the network of microorganisms that are linked with plants. These network models of soil and plant microbiomes can be interpreted for biocontrol and present new prospects for disease management. While single organisms were commonly utilized in the past, their effects were often uneven, microbiome-based biocontrol techniques are now possible (Berg et al., 2017; Jahagirdar et al., 2021). Microbial consortia offer numerous benefits for managing plant diseases compared to the application of a single strain such as i) Consortia of bio-control agents targets wide range of phytopathogens, ii) Enhancement of soil suppressiveness through increased microbial diversity within the soil rhizosphere, iii) Diverse species occupy different ecological niches in rhizosphere and thereby restricts competition between them, iv) Improved rhizosphere colonization due to interactions among microbes within the consortium, v) Utilization of multiple strains with varied modes of action, which boosts the bio-control efficiency of the consortia. Mixture of four bacterial isolates comprising of Serratia marcescens, Pseudomonas fluorescens, Rahnella aquatilis and Bacills amyloliquefaciens improved soil suppressiveness against Fusarium oxysporum f.sp. ciceris race 0 and Fusarium solani f.sp. pisi causing Fusarium decline in chickpea by increasing microbial diversity in rhizosphere (Palmieri et al., 2017). In the future, microbial consortia and biocontrol agents can be employed to improve biodiversity associated with crops via microbiome engineering to achieve definitive microbiome outcomes as desired (Erlacher et al., 2014; Jadav et al., 2022).
Microbiome engineering
Plant microbiome engineering can be achieved in two ways: bottoms-up methods involve isolating, altering, and reviving certain microbes, whereas top-down approaches involve synthetic ecology, which includes horizontal gene transfer to a variety of hosts in situ and then phenotyping the microbiome (Ab Rahman et al., 2018). The root microbiome can be engineered by soil conditioning techniques such as incorporating legumes to enrich native diazotrophic microorganisms. Additionally, the use of substrates/non-microbial biostimulants, including innovative organic soil amendments and root exudates, can help attract beneficial microbiota. Introducing external microbial inoculations, whether through soil application or as endophytes, can effectively manage Xanthomonas blight in rice (Saikia et al. 2020). Another strategy is to alter indigenous microbial strains through genetic engineering techniques, including CRISPR/Cas9 and RNA interference. This modification seeks to enhance the microbes' ability to withstand stress and optimize their nutrient utilization (Joshi et al. 2025). It would be extremely helpful to develop microbiomes that are long-lasting, stress-resistant, and capable of increasing agricultural output. Microbiome bioengineering is a fascinating choice for improving plant health and will provide enormous benefits to biocontrol methods in the future (Orozco-Mosqueda et al., 2018). Plant microbiome bioengineering is an intriguing option for improving a plant’s biological capabilities, an approach that, while still in its infancy, has the potential to be of immense agricultural value (del Carmen et al., 2018).
Genetically modified biocontrol agents
The recent advancements in genome engineering tools, genome-wide functional tools and meta-omic tools can improve our ability to design microbes for biocontrol and biofertilization, as well as increase agricultural productivity and yield. Rhizoctonia solani infection in beans can be brought under control by transferring a gene coding for the enzyme chitinase from Serratia to a Pseudomonas endophyte (Downing and Thomson, 2000). Clermont et al. (2011) employed genome shuffling to create superior Streptomyces melanosporofaciens EF76 biocontrol strains. Four strains with improved antagonistic activity against the potato diseases Streptomyces scabies and Phytophthora infestans were isolated after two rounds of genome shuffling. Biological control ability can also be improved by employing chemical mutagenesis. Examples include the use of nitrosoguanidine mutagenesis in Pseudomonas aurantiaca B-162 to produce a strain with better phenazine synthesis leading to improved biocontrol activity (Feklistova and Maksimova, 2008) and Trichoderma harzianum strains that exhibited enhanced biocontrol ability after UV mutagenesis (Marzano et al., 2013). A recombinant strain of Pseudomonas synxantha, designated ZHW 15 and ZHW 25, demonstrated enhanced biocontrol efficacy against soil-borne pathogens affecting wheat (Zhang et al. 2020). The addition of the required mutation can at times produce altered gene expression in non-targeted genes resulting in undesired effects. These constraints can be overcome using more recently established genome editing approaches. We can insert mutations into specific regions in the genome with high precision and efficiency using techniques like Crispr/Cas (Barrangou and van Pijkeren, 2016). The gene editing approach could also help in commercialization of BCA’s through ease of regulatory clearances.
Mycoviruses as biocontrol agents
Mycoviruses having capability to infect fungal pathogens are known to have the potential to be used as biological control agents against plant diseases. The mycoviruses were recognized to induce hypovirulence (reduced virulence) in their hosts and this notion elicited great interest in characterization of viruses from phytopathogenic fungi and to utilize them as biocontrol agents (García-Pedrajas et al., 2019). Surprisingly, numerous plant pathogenic fungi are found to harbor mycoviruses, which reduce the virulence of their fungal host (Kumar and Chandel, 2016). Hypovirulence-associated mycoviruses are those mycoviruses that reduce the pathogenicity of their inhabiting fungal hosts. However, it is difficult to transmit these mycoviruses easily between vegetatively incompatible groups and hence it has been difficult to develop commercial mycovirus biocontrol strategies for phytopathogenic fungi (Khalifa and MacDiarmid, 2021).
MAJOR CHALLENGES FOR BIOCONTROL RESEARCH IN THE PLANT DISEASE MANAGEMENT
Biological control strategy was initially employed at the end of the 19th century. Displaying an array of advantages, the literature has also mentioned many limitations of this biocontrol strategy for controlling plant diseases (Stenberg et al., 2021). Though these methods offer effective alternatives to chemical pesticides for controlling pests and plant diseases, there are still various challenges to overcome. These challenges are briefly discussed in the subsequent paragraphs.
The journey of biocontrol agents from lab to field
Researchers are screening potential microbes as part of their work to create biological pesticide remedies that are very effective for controlling plant diseases in agriculture. These strains are usually chosen based on factors such as disease resistance, host range, availability, formulation, mass production, and farmer practice (Farrar et al., 2014). Bioagents can be assessed by considering geographical locations, host crop species, soil types, and environmental factors. The growth of BCAs is typically easier to observe in controlled environments such as greenhouses. The preference of most researchers at this stage might be associated with the reliability of the environment (Spadaro and Gullino, 2004). The performance of BCAs in greenhouse trials can provide important theoretical and practical support for field utilization. The stability of BCAs is affected by the method of formulation, shipment, and storage environment. To achieve high levels of BCA strategy success, it is important to improve the formulation technology, extend the storage duration of the BCA product, optimize the production of selected microbial strains, and achieve large-scale application through low-cost manufacturing companies (Barratt et al., 2018). During the formulation and field efficacy trials, private sector partners and licensed laboratories conduct the essential environmental and human health assessments, as well as quality assurance for the ultimate commercialization of biopesticides. Before utilizing biopesticides on a large scale in the field, government authorities must grant permission. Typically, the biopesticide registration and regulation portfolio includes modified versions of synthetic pesticides as well as risk evaluation. This includes ecotoxicological and toxicological tests, as well as studies into their mechanism of action and host spectrum. Most of these requirements are difficult for authorities to achieve because producing effective bioagents while maintaining acceptable safety and consistency standards for commercialization might be a difficult task (Chandler et al., 2011).
Limited number of registered bioagents and lack of awareness
Despite their well-documented efficacy, BCAs now account for less than 5% of the crop protection sector’s commercial value (He et al., 2021). The identification, characterization, and registration of potential microbes takes more time and requires academic-industry collaborations (Stenberg et al., 2021). Furthermore, using natural resources to manage diseases (i.e., BCAs) raises several ethical and legal issues that might affect the biodiversity of an area (Sundh and Eilenberg, 2020). In this situation, new BCA species and populations have been restricted from entering specified countries. At present, every country has its own regulatory system, which varies widely. For example, high development costs for new commercial BCAs have been recognized as an obstacle to the BCA industry’s development in Australia (Hidayah, 2022). The standards for data submission for temporary registration in India are less demanding than those for standard registration. It is imperative to meet the quality standards set forth by the Central Insecticides Board, which encompass content, the virulence of the organism in terms of LC50, moisture content, shelf life, and secondary non-pathogenic microbial load. Biopesticides are exempted from the fees for pesticide registration and for the right to sell the product in Canada, except for the cost charged for reviewing the product label. Like Canada, the US does not require registration of indigenous beneficial insects or entomopathogenic nematodes or similar types of microbial inoculants as long as they do not make a pest control claim. In the U.S. and EU, biopesticide registration can take over 3 years as compared to 6-12 months for synthetic products. The timeline for obtaining approval and registration of a microbial pest control product, starting from the pre-consultation with the Pest Control Products Board, Kenya is influenced by the complexity of the product and generally ranges from 2 to 4 years (Hoeschle-Zeledon et al., 2013). To facilitate the registration and commercialization of novel BCAs and their products, BCA registration requires extensive cooperation among governmental institutes, universities, and industry sectors. The number of programs that provide financial and ecological benefits plays a vital role in bioagents registration (Stenberg et al., 2021). Hence, local usage and global marketing commercialization must fulfil international legislation. The International Biological Control Organization (IOBC) was established to bring together scholars, scientists, and professionals from a wide range of industries and fields to identify barriers and offer recommendations to overcome those (Brodeur et al., 2018). Biocontrol management has a direct impact on farmers’ expenses and income, but it also has an indirect impact on their financial benefits due to its effect on farmland biodiversity and environmental sustainability (Barratt et al., 2018). However, farmers have not fully utilized commercial bioagents due to a lack of information and awareness. Therefore, there is a need to strengthen the idea of biological management in the farming community to restore their trust in the use of bioagents (Ayaz et al., 2023; Hegde et al., 2023).
Bioagents commercialization and legislative procedure
Despite their growing popularity in crop disease management, biocontrol agents currently represent only 1% of agricultural control techniques, whereas synthetic-based pesticides account for 15% in the agriculture sector (Fravel, 2005). The commercialization of bioagents is a multi-step procedure that faces numerous challenges. Before being licensed for commercialization, similar to synthetic pesticides, BCAs are subjected to risk evaluations (Wilson and Jackson, 2013). The current trend of reducing the use of synthetic pesticides and simplifying the regulatory process for low-risk products may allow BCAs to be commercialized worldwide (Chandler et al., 2011; Hegde and Vijaykumar, 2022).
CONCLUSION AND PERSPECTIVES
Synthetic pesticides, due to their eco-toxicological risks, are facing increasing restrictions on their use worldwide. Biocontrol products based on microbial antagonists offer safer alternatives to replace or complement chemical pesticides. As a result, research on the selection, characterization and commercial development of BCAs is steadily increasing over the last few decades. Among the most studied biocontrol agents are fungi, bacteria and yeasts. Despite the large number of studies on biocontrol agents, the commercial formulations of BCA are still too limited and insufficient to meet the growing demand for sustainable agricultural systems. The main challenges hindering the development of new microbe-based formulation are (i) the sometimes lower efficacy of BCAs compared to synthetic pesticides, (ii) the lack of information available on the microbial formulation protocols, due to industrial secrecy and (iii) the complex registration and patent procedures in various countries. The use of molecular and omics tools could significantly improve the selection of biocontrol agents by providing a deeper understanding of their mechanisms of action, which is critical for optimizing BCAs activity and streamlining the registration processes. Future research should also investigate the economic feasibility and societal impact of expanding the use of biocontrol technologies. Analyzing the cost effectiveness and potential benefits for farmers and the agriculture sector would be valuable. For the widespread adoption of biocontrol strategies, it is essential to enhance dissemination among farmers, extension services, and policymakers. Efforts should focus on developing educational programs and outreach initiatives to raise awareness and make biocontrol solutions more accessible. Despite the technical and bureaucratic challenges associated with developing microbial BCAs, there is a growing shift towards plant disease control methods with lower environmental impact and fewer risks for human health. Additionally, political support for research into new technologies, particularly those addressing climate change and biodiversity conservation, is increasing. Consequently, research into the design and development of more efficient bioproducts, including microbial formulations to be used against plant diseases, will attract more and more attention in the near future.
REFERENCES
Ab Rahman, S. S. F., E. Singh, C. M. J. Pieterse and P. M. Schenk. 2018. Emerging microbial biocontrol strategies for plant pathogens. Plant Sci. 267: 102–111.
Agrios, G. N. 2005. Control of Plant Diseases. Academic Press: San Diego, CA, USA, pp. 293–353.
Arunkumar, K., S. R. Sivakumar and R. Rengasamy. 2010. Review on bioactive potential in seaweeds marine macroalgae: A special emphasis on bioactivity of seaweeds against plant pathogens. Asian J. Plant Sci. 9: 227–240.
Attai, H., J. Rimbey, G. P. Smith and P. J. B. Brown. 2017. Expression of a peptidoglycan hydrolase from lytic bacteriophages. Appl. Environ. Microbiol. 83: 14–17.
Ayaz, M., C. H. Li, Q. Ali, W. Zhao, Y. K. Chi, M. Shafiq, F. Ali, X. Y. Yu, Q. Yu, J. T. Zhao, et al. 2023. Bacterial and fungal biocontrol agents for plant disease protection: Journey from lab to field, current status, challenges, and global perspectives. Molecules. 28: 6735. https://doi.org/10.3390/molecules28186735.
Aziz, N. H., M. Z. El-Fouly, A. A. El-Essawy, M. A. Khalaf. 1997. Influence of bean seedling root exudates on the rhizosphere colonization by Trichoderma lignorum for the control of Rhizoctonia solani. Bot. Bull. Acad. Sin. 38: 33–39.
Bailly, A. and L. Weisskopf. 2017. Mining the volatilomes of plant-associated microbiota for new biocontrol solutions. Front. Microbiol. 8: 1638.
Baltruschat, H. and F. Schoenbeck. 1975. The influence of endotrophic mycorrhiza on the infestation on tobacco by Thielaviopsis basicola. Phytopath. Z 84: 172–188.
Barrangou, R. and J. P. van Pijkeren. 2016. Exploiting CRISPR-Cas immune systems for genome editing in bacteria. Curr. Opin. Biotechnol. 37: 61–68.
Barratt, B. I. P., V. C. Moran, F. Bigler and J. C. van Lenteren. 2018. The status of biological control and recommendations for improving uptake for the future. Bio Control. 63: 155–167.
Barrows-Broaddus, J. and T. J. Kerr. 1981. Inhibition of Fusarium moniliforme var. subglutinans, the causal agent of pine pitch canker, by the soil bacterium Arthrobacter sp. Can. J. Microbiol. 27: 20–27.
Batta, Y. A. 2004. Effect of treatment with Trichoderma harzianum Rifai formulated in invert emulsion on postharvest decay of apple blue mold. Int. J. Food Microbiol. 96: 281–288.
Berg, G., M. Köberl, D. Rybakova, H. Müller, R. Grosch and K. Smalla. 2017. Plant microbial diversity is suggested as the key to future biocontrol and health trends. FEMS Microbiol. Ecol. 93: 5.
Bisht, N., S. K. Mishra and P. S. Chauhan. 2020. Bacillus amyloliquefaciens inoculation alters physiology of rice (Oryza sativa L. var. IR-36) through modulating carbohydrate metabolism to mitigate stress induced by nutrient starvation. J. Biol. Macromol. 143: 937–951.
Bolwerk, A., A. L. Lagopodi, A. H. M. Wijfjes, G. E. M. Lamers, T. F. C. Chin-A-Woeng, J. J. Lugtenberg Ben and G. V. Bloemberg. 2003. Interactions in the tomato rhizosphere of two Pseudomonas biocontrol strains with the phytopathogenic fungus Fusarium oxysporum f. Sp. radicis-lycopersici. Mol. Plant-Microbe Interact. 16: 983–993.
Bonaterra, A., E. Badosa, N. Daranas, J. Frances, G. Rosello and E. Montesinos. 2022. Bacteria as biological control agents of plant diseases. Microorganisms. 10: 1759. https://doi.org/10.3390/microorganisms10091759.
Bora, L.C., R. Chetia, S. Saikia, and K. P. Baruah. 2018. Biopesticides: their role in sustainable agriculture, production technology and entrepreneurship opportunities in Assam. Dept. of Plant Pathology Assam Agricultural University. Jorhat, Assam.
Brewer, M. T. and R. P. Larkin. 2005. Efficacy of several potential biocontrol organisms against Rhizoctonia solani on potato. Crop Prot. 24: 939–950.
Burketova, L., L. Trda, , P. G. Ott and O. Valentova. 2015. Bio-based resistance inducers for sustainable plant protection against pathogens. Biotechnol. Adv., 33: 994–1004.
Cabrefiga, J., A. Bonaterra. and E. Montesinos. 2007. Mechanisms of antagonism of Pseudomonas fluorescens EPS62e against Erwinia amylovora, the causal agent of fire blight. Int. Microbiol. 10: 123–132.
Cazorla, F. M., S. B. Duckett, E. T. Bergstreom, S. Noreen, R. Odijk, B. J. Lugtenberg, J. E. Thomas-Oates and G. V. Bloemberg. 2006. Biocontrol of avocado dematophora root rot by antagonistic Pseudomonas fluorescens PCL1606 correlates with the production of 2-hexyl 5-propyl resorcinol. Mol. Plant-Microbe Interact. 19: 418–428.
Chan, Z. and S. Tian. 2005. Interaction of antagonistic yeasts against postharvest pathogens of apple fruit and possible mode of action. Postharvest Biol. Technol. 36: 215–223.
Chandler, D., A. S. Bailey, G. M. Tatchell, G. Davidson, J. Greaves and W. P. Grant. 2011. The development, regulation and use of biopesticides for integrated pest management. Philos. Trans. R. Soc. Lond. B Biol. Sci. 366: 1987–1998.
Chen, Y., Yunbao Wan, Xuefei Jiang, Qian Liu, Tao Wei and Maolin Wang. 2021. A novel fungal strain, Bjerkandera adusta BK-1, as a promising broad-spectrum biological control agent against plant fungal diseases. https://doi.org/10.1080/09583157.2021.1992347
Chernin, L., Z. Ismailov, S. Haran and I. Chet. 1995. Chitinolytic Enterobacter agglomerans antagonistic to fungal plant pathogens. Appl. Environ. Microbiol. 61: 1720–1726.
Chernin, L. S., L. Dela Fuente, V. Sobolev, S. Haran, C. E. Vorgias, A. B. Oppenheim, I. Chet. 1997. Molecular cloning, structural analysis, and expression in Escherichia coli of a chitinase gene from Enterobacter agglomerans. Appl. Environ. Microbiol. 63: 834–839.
Chowdhury, S. P., J. Uhl, R. Grosch, S. Alqueres, S. Pittroff, K. Dietel, P. Schmitt-Kopplin, R. Borriss and A. Hartmann. 2015. Cyclic lipopeptides of Bacillus amyloliquefaciens subsp. plantarum colonizing the lettuce rhizosphere enhance plant defense responses toward the bottom rot pathogen Rhizoctonia solani. Mol. Plant Microbe Interact. 28: 984–995.
Clarkson J. P., T. Payne, A. Mead and J. M. Whipps. 2002. Selection of fungal biological control agents of Sclerotium cepivorum for control of white rot by sclerotial degradation in a UK soil. Plant Pathol. 51: 735–745.
Clermont, N., S. Lerat and C. Beaulieu. 2011. Genome shuffling enhances biocontrol abilities of Streptomyces strains against two potato pathogens. J. Appl. Microbiol. 111: 671–682.
Coombs, J. T., P. P. Michelsen and C. M. M Franco. 2004. Evaluation of endophytic actinobacteria as antagonists of Gaeumannomyces graminis var. tritici in wheat. Biol. Control. 29: 359–366.
Cotxarrera, L., M, I, Trillas-Gay, C, Steinberg and C. Alabouvette. 2002. Use of sewage sludge compost and Trichoderma asperellum isolates to suppress Fusarium wilt of tomato. Soil Biol. Biochem. 34: 467–476.
Daranas, N., G. Rosello, J. Cabrefiga, I. Donati, J. Frances, E. Badosa, F. Spinelli, E. Montesinos and A. Bonaterra. 2019. Biological control of bacterial plant diseases with Lactobacillus plantarum strains selected for their broad-spectrum activity. Ann. Appl. Biol. 174: 92–105.
de Boer, M., P. Bom, F. Kindt, J. J. B. Keurentjes, I. van der Sluis, L. C. van Loon and P. A. H. M. Bakker. 2003. Control of Fusarium wilt of radish by combining Pseudomonas putida strains that have different disease-suppressive mechanisms. Biol. Control. 93: 626–632.
del Carmen, M., M. del Carmen Rocha-Granados and G. Glick. 2018. Microbiome engineering to improve biocontrol and plant growth promoting mechanisms. Microbiol. Res. 208: 25–31.
Di Giallonardo, F. and E. C. Holmes. 2015. Viral biocontrol: Grand experiments in disease emergence and evolution. Trends Microbiol. 23: 83–90. https://doi.org/10.1016/j.tim.2014.10.004
Diaz-Diaz, M., A. Bernal-Cabrera, A. Trapero, R. Medina-Marrero, S. Sifontes-Rodríguez, R. D. Cupull-Santana, M. García-Bernal and C. Agustí-Brisach. 2022. Characterization of actinobacterial strains as potential biocontrol agents against Macrophomina phaseolina and Rhizoctonia solani, the main soil-borne pathogens of Phaseolus vulgaris in Cuba. Plants. 11: 645.
Dobhal, A. and G. M. Hegde. 2021. Shelf life study of Pseudomonas fluorescens in liquid formulations. J. Farm Sci. 34(1): 84-86.
Downing, K. and J. A. Thomson. 2000. Introduction of the Serratia marcescens chiA 671 gene into an endophytic Pseudomonas fluorescens for the biocontrol of phytopathogenic fungi. Can. J. Microbiol. 46: 363–369.
Droby, S., E. Chalutz, R. Hofstein, C. L. Wilson, M. E. Wisniewski, B. Fridlender, L. Cohen, B. Weiss and A. Daus. 1993. Pilot testing of Pichia guilliermondii: a biocontrol agent of postharvest diseases of citrus fruit. Biol. Control. 3: 47–52.
Dunne, C., Y. Moenne-Loccoz, F. J. de Bruijin and F. O’Gara. 2000. Overproduction of an inducible extracellular serine protease improves biological control of Pythium ultimum by Stenotrophomonas maltophilia strain W81. Microbiology. 146: 2069–2078.
Dy, R. L., L. A. Rigano and P. C. Fineran. 2018. Phage-based biocontrol strategies and their application in agriculture and aquaculture. Biochem. Soc. Trans. 46: 1605–1613.
El-Tarabily, K. A. 2003. An endophytic chitinase-producing isolate of Actinoplanes missouriensis, with potential for biological control of root rot of lupine caused by Plectosporium tabacinum. Aust. J. Bot. 51: 257–266.
El-Tarabily, K. A., N. H. Soliman, A. H. Nassar, H. A. Al-Hassani, K. Sivasithamparam, F. Mc Kenna and G. E. Hardy. 2000. Biological control of Sclerotinia minor using a chitinolytic bacterium and actinomycetes. Plant Pathol. 49: 576–583.
El-Tarabily, K. A. 2006. Rhizosphere-competent isolates of streptomycete and non-streptomycete actinomycetes capable of producing cell-wall degrading enzymes to control Pythium aphanidermatum damping-off disease of cucumber. Can. J. Bot. 84: 211–222.
El-Tarabily, K. A., G. E. S. J. Hardy, K. Sivasithamparam, A. M. Hussein and I. D. KurtboE` ke. 1997. The potential for the biological control of cavity spot disease of carrots caused by Pythium coloratum by streptomycete and non-streptomycete actinomycetes in Western Australia. New Phytol. 137: 495–507.
Escande, A. R., F. S. Laich and M. V. Pedraza. 2002. Field testing of honeybee dispersed Trichoderma spp. to manage sunflower head rot (Sclerotinia sclerotiorum). Plant Pathol. 51: 346–351.
Esserti, S., A. Smaili, L. A. Rifai, T. Koussa, K. Makroum, M. Belfaiza, E. M. Kabil, L. Faize, L. Burgos and N. Alburquerque. 2017. Protective effect of three brown seaweed extracts against fungal and bacterial diseases of tomato. J. Appl. Phycol. 29: 1081–1093.
Fan, B., J. Blom, H. P. Klenk and R. Borriss. 2017. Bacillus amyloliquefaciens, Bacillus velezensis and Bacillus siamensis form an operational Group B. amyloliquefaciens within the B. subtilis species complex. Front Microbiol. 8: 22. https://doi.org/10.3389/fmicb.2017.00022
Farrar, K., D. Bryant and N. Cope-Selby. 2014. Understanding and engineering beneficial plant-microbe interactions: Plant growth promotion in energy crops. Plant Biotechnol. J. 12: 1193–1206.
Feklistova, I. N. and N. P. Maksimova. 2008. Obtaining Pseudomonas aurantiaca strains capable of overproduction of phenazine antibiotics. Microbiology. 77: 176–180.
Folimonova, S. Y. 2013. Developing an understanding of cross-protection by Citrus tristeza virus. Front Microbiol. 4: 76.
Folman, L. B., J. Postma and J. A. Van Veen. 2003. Inability to find consistent bacterial biocontrol agents of Pythium aphanidermatum in cucumber using screens based on ecophysiological traits. Microb. Ecol. 45: 72–87.
Fravel, D. R. 2005. Commercialization and implementation of biocontrol 1. Annu. Rev. Phytopathol. 43: 337–359.
García-Pedrajas, M. D., M. C. Cañizares, J. L. Sarmiento-Villamil, A. G. Jacquat and J. S. Dambolena. 2019. Mycoviruses in biological control: From basic research to field implementation. Phytopathology. 109: 1828–1839.
Ghorbanpour, M., M. Omidvari, P. Abbaszadeh-Dahaji, R. Omidvar and K. Kariman. 2018. Mechanisms underlying the protective effects of beneficial fungi against plant diseases. Biol. Control. 117: 147–157.
Hanson, L. E. 2000. Reduction of Verticillium wilt symptoms in cotton following seed treatment with Trichoderma virens. J. Cotton Sci. 4: 224–231.
He, D. C., M. H. He, D. M. Amalin, W. Liu, D. G. Alvindia and J. Zhan, 2021. Biological control of plant diseases: An evolutionary and eco-economic consideration. Pathogens. 10: 1311. https://doi.org/10.3390/pathogens10101311.
Hegde G. M. and L. H. Malligawad. 2020. Bioagents for the management of mildews in cucumber under protected cultivation. J. Mycol. Plant Pathol. 50(1): 1–10.
Hegde, G. M. and K. N. Vijaykumar. 2022. Formulation, application and commercialization of biopesticides in india. Asia Pacific Biofertilizers and Biopesticides Information Platform (APBB). https://apbb.fftc.org.tw/article/306. pp. 1-25.
Hegde, G. M. and K. N. Vijaykumar. 2023. Mechanisms of resistance of Trichoderma spp. against plant disease management. Asia Pacific Biofertilizers and Biopesticides Information Platform (APBB). https://apbb.fftc.org.tw/article/413 pp. 1-15.
Hegde, G. M. and S. Jahagirdar. 2020. Trichoderma: A potential millennium microbe for sustainable management of field and horticulture crop diseases: An overview. J. Farm Sci. 33(2): 159-172.
Hegde, G. M., A. Dobhal, K. N. Vijaykumar and S. Jahagirdar. 2023. Advances in formulations and efficacy of mycopesticides for plant disease management and sustainable yields. In: Fungal Resources for Sustainable Economy. Singh, I., V. R. Rajpal and S. S. Navi (eds) Springer, Singapore. https:/doi.org/10.1007/978-981-19-9103-5_14
Hegde, G. M., L. H. Malligawad, M. N. Sreenivasa and B. K. Chetri. 2022. Role of plant growth promoting microbes in the control of fungal foliar diseases of tomato under protected cultivation. Egypt. J. Biol. Pest Control. 32: 105. https://doi.org/10.1186/s41938-022-00606-7
Heungens, K. and J. Parke. 2000. Zoospore homing and infection events: effects of the biocontrol bacterium Burkholderia cepacia AMMDR1 on two oomycete pathogens of pea (Pisum sativum L.). Appl. Environ. Microbiol. 66: 5192–5200.
Hidayah, B. N. 2022. Biological control potential of Trichoderma species and bacterial antagonists against Sclerotinia sclerotiorum on canola in Western Australia. Int. J. Agric. Biol. 27: 215–227.
Hoeschle-Zeledon, I., P. Neuenschwander and L. Kumar. 2013. Regulatory challenges for biological control. SP-IPM Secretariat, International Institute of Tropical Agriculture (IITA), Ibadan, Nigeria. p. 43.
Hu, H., M. E. Wisniewski, A. Abdelfattah and X. Zheng. 2017. Biocontrol activity of a cold-adapted yeast from Tibet against grey mold in cherry tomato and its action mechanism. Extremophiles. 21: 789–803.
Hunziker, L., D. Beonisch, U. Groenhagen, A. Bailly, S. Schulz and L. Weisskopf. 2015. Pseudomonas strains naturally associated with potato plants produce volatiles with high potential for inhibition of Phytophthora infestans. Appl. Environ. Microbiol. 81: 821–830.
Ikeda, S., A. Shimizu, M. Shimizu, H. Takahashi and S. Takenaka. 2012. Biocontrol of black scurf on potato by seed tuber treatment with Pythium oligandrum. Biol. Control. 60: 297–304.
Iqbal, S. H., K. S. Qureshi and S. Ahmed. 1977. Influence of VA mycorrhiza on damping-off caused by Rhizoctonia solani in Brassica napus. Biologia. 23: 197–208.
Jadav, P. C., G. M. Hegde, S. Jahagirdar and V. Navi. 2022. Evaluation of microbial consortia against Sclerotium rolfsii Sacc. causing foot rot of wheat. J. Farm Sci. 35(2): 219-222.
Jahagirdar, S., G. M. Hegde, P. U. Krishnaraj and D. N. Kambrekar. 2021. Microbial consortia for plant disease management and sustainable productivity. In: Emerging Trends in Plant Pathology. Singh, K. P., S. Jahagirdar and B. K. Sarma (eds). Springer Nature Singapore Pvt. Ltd. https://doi.org/10.1007/978-981-15-6275-4
Jiang, Z., Y. Guo., S. Li, H. Qi and J. Guo. 2006. Evaluation of biocontrol efficiency of different Bacillus preparations and field application methods against Phytophthora blight of bell pepper. Biol. Control. 36: 216–223.
Jimenez, E., F. Dorta, C. Medina, A. Ramirez and I. Ramirez. 2011. Pena-Cortes, H. Anti-pathogenic activities of macro-algae extracts. Mar. Drugs. 9: 739–756.
Joshi, D., A. Kaushik, R. Kumar, A. Arya, G. Santoyo, V. K. Singh, N. Kashyap, M. K. Solanki, M. Kumari, N. Bhardwaj and A. Kumar. 2025. Improving plant performance through microbiome manipulation: The potential role of current bioengineering approaches. Bacteria. 4(1): 12.
Kamilova F, J. H. J. Leveau and B. J. J. Lugtenberg. 2007. Collimonas fungivorans, an unpredicted in vitro but efficient in vivo biocontrol agent for the suppression of tomato foot and root rot. Environ. Microbiol. 9: 597–1603.
Kasun, M., A. Dinushani, J. L. Alan, S. Phillips, D. Kannangara and P. Itthayakorn. 2020. Fungi vs fungi in biocontrol: An overview of fungal antagonists applied against fungal plant pathogens. Front. Cell. Infect. Microbiol. 10. https://doi.org/10.3389/fcimb.2020.604923
Kavino, M., S. Harish, N. Kumar, D. Saravanakumar and R. Samiyappan. 2008. Induction of systemic resistance in banana (Musa spp.) against Banana bunchy top virus (BBTV) by combining chitin with root-colonizing Pseudomonas fluorescens strain CHA0. Eur. J. Plant Pathol. 120: 353–362.
Khalifa, M. E. and R. M. MacDiarmid. 2021. A mechanically transmitted DNA mycovirus Is targeted by the defence machinery of its host, Botrytis cinerea. Viruses. 13: 1315.
Köhl, J., R. Kolnaar and W. J. Ravensberg. 2019. Mode of action of microbial biological control agents against plant diseases: Relevance beyond efficacy. Front. Plant Sci. 10: 845. doi: 10.3389/fpls.2019.00845.
Konstantinidou-Doltsinis, S., E. Markellou, N. Petsikos-Panayotarou, E. Siranidou, A. E. Kalamarakis, A. Schmitt, A. Ernst, B. Seddon, R. R. Belanger, A. J. Dik, D. Shtienberg. 2002. Biological control of fungal and bacterial plant pathogens. In: Combinations of biocontrol agents and Milsana(R) against powdery mildew and grey mould in cucumber in Greece and the Netherlands. Elad Y. and J. Kohl (eds). Vol 25. IOBC-WPRS Working Group, Kusadasi, pp. 171–174.
Koumoutsi, A., X. H. Chen, A. Henne, H. Liesegang, G. Hitzeroth, P. Franke, J. Vater and R. Borriss. 2004. Structural and functional characterization of gene clusters directing nonribosomal synthesis of bioactive cyclic lipopeptides in Bacillus amyloliquefaciens strain FZB42. J. Bacteriol. 186: 1084–1096.
Krishna, K. R. and D. J. Bagyaraj. 1983. Interaction between Glomus fasciculatus and Sclerotium rolfsii in peanut. Can. J. Bot. 67: 2349–2357.
Kumar, A., A. K. Chaturvedi, K. Yadav, K. P. Arunkumar, S. K. Malyan, P. Raja and A. N. Yadav. 2019. Fungal phytoremediation of heavy metal-contaminated resources: current scenario and future prospects. In: Recent Advancement. In: White Biotechnology Through Fungi. 437-461, Springer.
Kumar, V. and S. Chandel. 2016. Mycoviruses and their role in biological control of plant diseases. Int. J. Plant Sci. 11: 375–382.
Lahlali, R., S. Ezrari, N. Radouane, J. Kenfaoui, Q. Esmaeel, H. El Hamss, Z. Belabess and E. A. Barka. 2022. Biological control of plant pathogens: A global perspective. microorganisms, 10: 596. https://doi.org/10.3390/microorganisms10030596.
Lakkis, S., P. Trotel-Aziz, F. Rabenoelina, A. Schwarzenberg, E. Nguema-Ona, C. Clement and A. Aziz. 2019. Strengthening grapevine resistance by Pseudomonas fluorescens PTA-CT2 relies on distinct defense pathways in susceptible and partially resistant genotypes to downy mildew and grey mold diseases. Front. Plant Sci. 10: 1112.
Lecoq H. and J. M. Lemaire. 1991. Control of Zucchini yellow mosaic virus in squash by cross protection. Plant Dis. 75: 208–211.
Lherminier, J., N. Benhamou, J. Larrue, M. L. Milat, E. Boudon-Padieu, M. Nicole and J. P. Blein. 2003. Cytological characterization of elicitin-induced protection in tobacco plants infected by Phytophthora parasitica or Phytoplasma. Phytopathology. 93: 1308–1319.
Li, S., C. C. Jochum, F. Yu, K. Zaleta-Rivera, L. Du, S. D. Harris and G. Y. Yuen. 2008. An antibiotic complex from Lysobacter enzymogenes strain C3: antimicrobial activity and role in plant disease control. Phytopathology. 98: 695–701.
Lou, B. G., A. Y. Wang, C. Lin, T. Xu and X. D. Zheng. 2011. Enhancement of defense responses by oligandrin against Botrytis cinerea in tomatoes. Afr. J. Biotechnol. 10: 442–449.
Madsen, A. M. and E. de Neergaard. 1999. Interactions between the mycoparasite Pythium oligandrum and sclerotia of the plant pathogen Sclerotinia sclerotiorum. Eur. J. Plant Pathol. 105: 761–768.
Marco, J. L. D., M. C. Valadares-Inglis and C. R. Felix. 2003. Production of hydrolytic enzymes by Trichoderma isolates with antagonistic activity against Crinipellis perniciosa, the causal agent of witches’ broom of cocoa. Braz. J. Microbiol. 34: 33–38.
Marzano, M., A, Gallo and C. Altomare. 2013. Improvement of biocontrol efficacy of Trichoderma harzianum vs. Fusarium oxysporum f. sp. lycopersici through UV-induced tolerance to fusaric acid. Biol. Control. 67: 397–408.
Mateos, E., J. Olaizola, J. A. Pajares, V. Pando and J. J. Diez. 2017. Influence of Suillus luteus on Fusarium damping-off in pine seedlings. African J. Biotechnol. 16(6): 268-273.
Mishra, S., A. Singh, C. Keswani, A. Saxena, B. K. Sarma and H. B. Singh. 2015. Harnessing plant-microbe interactions for enhanced protection against phytopathogens. In plant microbes Symbiosis: Applied Facets. Springer, New Delhi, India, pp. 111–125.
Mishra, V., W. Ellouze and R. Howard. 2018. Utility of arbuscular mycorrhizal fungi for improved production and disease mitigation in organic and hydroponic greenhouse crops. J. Hortic. 5(3): 1000237. DOI:10.4172/2376-0354.1000237
Mouloud, G., H. Daoud, J. Bassem, I, Laribi Atef and B. Hani. 2013. New bacteriocin from Bacillus clausii strain GM17: Purification, characterization, and biological activity. Appl. Biochem. Biotechnol. 171: 2186–2200.
Murphy, J. F., G. W. Zehnder, D. J. Schuster, E. J. Sikora, J. E. Polston and J. W. Kloepper. 2000. Plant growth promoting rhizobacterial mediated protection in tomato against Tomato mottle virus. Plant Dis. 84: 779–784.
Naureen, Z., A. H. Price, F. Y. Hafeez and M. R. Roberts. 2009. Identification of rice blast disease suppressing bacterial strains from the rhizosphere of rice grown in Pakistan. Crop Prot. 28: 1052–1060.
Niranjan Raj, S., S. A. Deepak, P. Basavaraju, H. S. Shetty, M. S. Reddy and J. W. Kloepper. 2003. Comparative performance of formulations of plant growth promoting rhizobacteria in growth promotion and suppression of downy mildew in pearl millet. Crop Prot. 22: 579–588.
Orozco-Mosqueda, M. D. C., M. D. C. Rocha-Granados, B. R. Glick and G. Santoyo. 2018. Microbiome engineering to improve biocontrol and plant growth-promoting mechanisms. Microbiol. Res. 208: 25–31.
Osman, M. E. H., M. M. El-Sheekh, A. M. Metwally, I. Aea and M. Ismail. 2011. Antagonistic activity of some fungi and cyanobacteria species against Rhizoctonia solani. Int. J. Plant Pathol. 2: 101–114.
Palmieri, D., D. Vitullo, F. De Curtis and G. Lima, 2017, A microbial consortium in the rhizosphere as a new biocontrol approach against Fusarium decline of chickpea. Plant and Soil. 412: 425-439.
Palmieri, D., G. Barone, R. A. Cigliano, F. De Curtis, G. Lima, R. Castoria and G. Ianiri. 2021. Complete genome sequence of the biocontrol yeast Papiliotrema terrestris strain LS28. G3 Genes|Genomes|Genetics. 11: 332.
Pandit, M. A., J. Kumar, S. Gulati, N. Bhandari, P. Mehta, R. Katyal, C. D. Rawat, V. Mishra and J. Kaur. 2022. Major biological control strategies for plant pathogens. Pathogens. 11: 273. https://doi.org/10.3390/pathogens11020273
Parasuraman, P., S. Pattnaik and S. Busi. 2019. Phyllosphere microbiome: Functional importance in sustainable agriculture. In New and Future Developments in Microbial Biotechnology and Bioengineering. Singh, J. S. and D. P. Singh. Eds.; Elsevier: Amsterdam, The Netherlands, Chapter 10; pp. 135–148. ISBN 978-0-444-64191-5.
Peshin, R, R. S. Bandral, W. J. Zhang, et al. 2009. Integrated Pest Management: A Global Overview of History, Programs and Adoption. In: Integrated Pest Management: Innovation-Development Process (Vol. 1) (Peshin R, Dhawan AK, eds). 1-50, Springer, Netherlands.
Peshin, R. and W. J. Zhang. 2014. Integrated pest management and pesticide use. In: Integrated Pest Management: Pesticide Problems. Vol. 3. (eds, Pimentel, D. and R. Peshin). pp. 1-46. Springer, Netherlands.
Picard, K., M. Ponchet, J. P. Blein, P. Rey, Y. Tirilly and N. Benhamou. 2000. Oligandrin. A proteinaceous molecule produced by the mycoparasite Pythium oligandrum induces resistance to Phytophthora parasitica infection in tomato plants. Plant Physiol. 124: 379–395.
Pliego, C., S. de Weert, G. Lamers, A. de Vicente, G. V. Bloemberg, F. M. Cazorla and C. Ramos. 2008. Two similar enhanced root colonizing Pseudomonas strains differ largely in their colonization strategies of avocado roots and Rosellinia necatrix hyphae. Environ. Microbiol. 10: 3295–3304.
Prashant, P., Jambhulkar, P. Sharma and R. Yadav. 2016. Delivery systems for introduction of microbial inoculants in the field. In: Microbial Inoculants in Sustainable Agricultural Productivity. (eds. Singh D. P., et al.,). pp. 199–218.
Qu, Z., Y. Fu, Y. Lin, Z. Zhao, X. Zhang, J. Cheng, J. Xie, T. Chen, B. Li and D. Jiang. 2021. Transcriptional responses of Sclerotinia sclerotiorum to the infection by SsHADV-1. J. Fungi. 7: 493.
Ram, R. M., C. Keswani, K. Bisen, R. Tripathi, S. P. Singh and H. B. Singh. 2018. Biocontrol technology: Eco-friendly approaches for sustainable agriculture. In: Omics Technologies and Bio-Engineering. Academic Press. Cambridge, MA, USA, pp. 177–190.
Rekanovic, E., S. Milijasevic, B. Todorovic and I. Potocnik. 2007. Possibilities of biological and chemical control of Verticillium wilt in pepper. Phytoparasitica. 35: 436–441.
Risoli, S., L. Cotrozzi, S. Sarrocco, M. Nuzzaci, E. Pellegrini and A. Vitti. 2022. Trichoderma-induced resistance to Botrytis cinerea in Solanum species: A meta-analysis. Plants. 11: 180.
Roberti, R., S. Galletti, P. L. Burzi, H. Righini, S. Cetrullo and C. Perez. 2015. Induction of defence responses in zucchini (Cucurbita pepo) by Anabaena sp. water extract. Biol. Control. 82: 61–68.
Roca-Couso, R., J. D. Flores-Felix and R. Rivas. 2021. Mechanisms of action of microbial biocontrol agents against Botrytis cinerea. J. Fungi. 12: 1045.
https://doi.org/10.3390/jof7121045
Saikia, K., L. C. Bora, P. Bora and H. Hazarika. 2020. Management of bacterial blight in rice (Oryza sativa) through combined application of endophytes and rhizosphere antagonist. Indian J. Agric. Sci. 90(12): 2323–2327.
Saravanakumar, D., N. Lavanya, K. Muthumeena, T. Raguchander and R. Samiyappan. 2009. Fluorescent pseudomonad mixtures mediate disease resistance in rice plants against sheath rot (Sarocladium oryzae) disease. BioControl. 54: 273.
Savary, S., L. Willocquet, S. J. Pethybridge, P. Esker, N. McRoberts and A. Nelson. 2019. The global burden of pathogens and pests on major food crops. Nat. Ecol. Evol. 3: 430–439.
Saxena, A. K., H. Chakdar, M. Kumar, et al. 2021. ICAR technologies: Biopesticides for eco-friendly pest management. Indian Council of Agricultural Research, New Delhi.
Saxena, A. K., M. Kumar, H. Chakdar, N. Anuroopa and D. J. Bagyaraj. 2020. Bacillus species in soil as a natural resource for plant health and nutrition. J. Appl. Microbiol. 128: 1583–1594. https://doi.org/10.1111/jam.14506.
Silva, H. S. A., R. S. Romeiro, R. Carrer-Filho, J. L. A. Pereira, E. S. G. Mizubuti and A. Mounteer. 2004. Induction of systemic resistance by Bacillus cereus against tomato foliar diseases under field conditions. J. Phytopathol. 152: 371–375.
Simon, A. 1989. Biological control of take-all of wheat by Trichoderma koningii under controlled environmental conditions. Soil Biol. Biochem. 21: 323–326.
Singh, I. 2017. Arbuscular mycorrhiza mediated control of plant pathogens. In: Mycorrhiza—Nutrient Uptake, Biocontrol, Ecorestoration. (Varma, A., R. Prasad, and N. Tuteja. eds.) Springer, Cham, Switzerland.
Smith, G. E. 1957. Inhibition of Fusarium oxysporum f.sp. lycopersici by a species of Micromonospora isolated from tomato. Phytopathology. 47: 429–432.
Spadaro, D. and M. L. Gullino. 2004. State of the art and future prospects of the biological control of postharvest fruit diseases. Int. J. Food Microbiol. 91: 185–194.
Stenberg, J. A., I. Sundh, P. G. Becher, C. Bjorkman, M. Dubey, P. A. Egan, H. Friberg, J. F. Gil, D. F. Jensen, M. Jonsson, M. Karlsson, S. Khalil, V. Ninkovic, G. Rehermann, R. R. Vetukuri and M. Viketoft. 2021. When is it biological control? A framework of definitions, mechanisms, and classifications. J. Pest Sci. 94: 665–676.
https://doi.org/10.1007/s10340-021-01354-7
Sugiprihatini, D., S. Wiyono and S. Widodo. 2011. Selection of yeasts antagonists as biocontrol agent of mango fruit rot caused by Botryodiplodia theobromae. Microbiol Indones. 5: 154–159.
Sundh, I. and J. Eilenberg. 2020. Why has the authorization of microbial biological control agents been slower in the EU than in comparable jurisdictions. Pest Manag. Sci. 77: 2170–2178.
Swain, H., T. Adak, A. K. Mukherjee, P. K. Mukherjee, P. Bhattacharyya, S. Behera, T. B. Bagchi, R. P. Shasmita, A. Khandual, M. K. Bag, T. K. Dangar, S. Lenka and M. Jena. 2018. Novel Trichoderma strains isolated from tree barks as potential biocontrol agents and biofertilizers for direct seeded rice. Microbiol. Res. 214: 83-90. https://doi.org/10.1016/j.micres.2018.05.015.
Tahir, H. A. S., Q. Gu, H. Wu, Y. Niu, R. Huo and X. Gao. 2017. Bacillus volatiles adversely affect the physiology and ultra-structure of Ralstonia solanacearum and induce systemic resistance in tobacco against bacterial wilt. Sci. Rep. 7: 40481.
Takenaka, S. and S. Ishikawa. 2013. Biocontrol of sugar beet seedling and taproot diseases caused by Aphanomyces cochlioides by Pythium oligandrum treatments before transplanting. Jpn. Agric. Res. Quarter. 47: 75–83.
Takenaka, S. and H. Tamagake. 2009. Foliar spray of a cell wall protein fraction from the biocontrol agent Pythium oligandrum induces defence-related genes and increases resistance against Cercospora leaf spot in sugar beet. J. Gen. Plant Pathol. 75: 340–348.
Tennant, P. F., C. Gonsalves, K. S. Ling, M. Fitch, R. Manshardt, J. L. Slinghtom and D. Gonsalves. 1994. Differential protection against Papaya ring spot virus isolates in coat protein gene transgenic papaya and classically cross-protected papaya. Phytopathology. 84: 1359–1366.
Tilocca, B., A. Cao and Q. Migheli. 2020. Scent of a killer: Microbial volatilome and its role in the biological control of plant pathogens. Front. Microbiol. 11: 41.
Trappe, J. M. 1962. Fungus association of ectotrophic mycorrhizae. Bot. Rev. 28: 538-606.
Ugras, S., K. Sezen, H. Kati and Z. Demirbag. 2013. Purification and characterization of the bacteriocin Thuricin Bn1 produced by Bacillus thuringiensis subsp. kurstaki Bn1 isolated from a hazelnut pest. J. Microbiol. Biotechnol. 23: 167–176.
Vallance, J., G. Le Floch, F. Deniel, G. Barbier, C. A. Levesque and P. Rey. 2009. Influence of Pythium oligandrum biocontrol on fungal and oomycete population dynamics in the rhizosphere. Appl. Environ. Microbiol. 75: 4790–4800.
Veresoglou, S. D and M. C. Rillig. 2012. Suppression of fungal and nematode plant pathogens through arbuscular mycorrhizal fungi. Biol. Lett. 8: 214–217.
Vicedo, B., R. Penalver, M. J. Asins and M. M. Lopez. 1993. Biological control of Agrobacterium tumefaciens, colonization, and pAgK84 transfer with Agrobacterium radiobacter K84 and the Tra- mutant strain K1026. Appl. Environ. Microbiol. 59: 309–315.
Walterson, A. M. and J. Stavrinides. 2015. Pantoea: Insights into a highly versatile and diverse genus within the Enterobacteriaceae. FEMS Microbiol. Rev. 39: 968–984.
Walther, D. and D. Gindrat. 1988. Biological control of damping-off of sugar-beet and cotton with Chaetomium globosum or a fluorescent Pseudomonas sp. Can. J. Microbiol. 34: 631–637.
Weiss, A., G. Mögel and S. Kunz. 2006. Development of “Boni-Pro-tect”-a Yeast Preparation for Use in the Control of Post-harvest Diseases of Apples. In: Proceedings of the 12th International Conference on Cultivation Technique and Phytopathological Problems in Organic Fruit-Growing, Weinsberg, Germany, 31st January–2nd February 2006.
Wilson, M. J. and T. A. Jackson. 2013. Progress in the commercialisation of bionematicides. BioControl. 58: 715–722.
Wisniewski, M., C. Biles, S. Droby, R. McLaughlin, C. Wilson and E. Chalutz. 1991. Mode of action of the postharvest biocontrol yeast, Pichia guilliermondii. I. characterization of attachment to Botrytis cinerea. Physiol. Mol. Plant Pathol. 39: 245–258.
Wittmann, J., C. Brancato, K. W. Berendzen and B. Dreiseikelmann. 2016. Development of a tomato plant resistant to Clavibacter michiganensis using the endolysin gene of bacteriophage CMP1 as a transgene. Plant Pathol. 65: 496–502.
www.custommarketinsight.com
Xiao, K., L, Kinkel and D. A. Samac. 2002. Biological control of Phytophthora root rots on alfalfa and soybean with Streptomyces. Biol Control. 23: 285–295.
Xue, Y., Y. Zhang, K. Huang, X. Wang, M. Xing, Q. Xu and Y. Guo. 2023. A novel biocontrol agent Bacillus velezensis K01 for management of grey mold caused by Botrytis cinerea. AMB Express. 13: 91. doi: 10.1186/s13568-023-01596-x. PMID: 37642883; PMCID: PMC10465465.
Yao, H. J. and S. P. Tian. 2005. Effect of pre- and post-harvest application of salicylic acid or methyl jasmonate on inducing disease resistance of sweet cherry fruit in storage. Postharvest Biol. Technol. 35: 253–262.
You, M. P., K. Sivasithamparam, D. I. Kurtbeoke. 1996. Actinomycetes in organic mulch used in avocado plantations and their ability to suppress Phytophthora cinnamomi. Biol. Fertil. Soils. 22: 237–242.
Zehnder, G. W., J. F. Murphy, E. J. Sikora and J. W. Kloepper. 2001. Application of rhizobacteria for induced resistance. Eur. J. Plant Pathol. 107: 39–50.
Zehnder, G.W., C. Yao, J. F. Murphy, E. J. Sikora and J. W. Kloepper. 2000. Induction of resistance in tomato against cucumber mosaic cucumovirus by plant growth-promoting rhizobacteria. BioControl. 45: 127–137.
Zhang, J., D. V. Mavrodi, M. Yang, L. S. Thomashow, O. V. Mavrodi, J. Kelton and D. M. Weller. 2020. Pseudomonas synxantha 2–79 transformed with pyrrolnitrin biosynthesis genes has improved biocontrol activity against soilborne pathogens of wheat and canola. Phytopathol. 110: 1010–1017.
Zhang, S., A. L. Moyne, M. S. Reddy and J. W. Kloepper. 2002. The role of salicylic acid in induced systemic resistance elicited by plant growth-promoting rhizobacteria against blue mold of tobacco. Biol. Control. 25: 288–296.
Zhang, S., T. L. White, M. C. Martinez, J. A. McInroy, J. W. Kloepper and W. Klassen. 2010. Evaluation of plant growth-promoting rhizobacteria for control of Phytophthora blight on squash under greenhouse conditions. Biol. Control. 53: 129–135.
Zhang, W. J. 2018. Global pesticide use: Profile, trend, cost / benefit and more. Proc. Int. Acad. Ecol. Environ. Sci. 8(1): 1–27.
Zhang, W. J., F. B. Jiang and J. F. Ou. 2011. Global pesticide consumption and pollution: with China as a focus. Proc. Int. Acad. Ecol. Environ. Sci. 1(2): 125–144
Advances, Challenges and Perspectives in the Application of Novel Biocontrol Agents for Plant Disease Management
DOI: https://doi.org/10.56669/HYZL2696
ABSTRACT
The growing concerns among consumers and governments regarding the issues associated with synthetic chemicals in disease control and food safety have prompted growers to seek eco-friendly alternatives to traditional chemical-based practices. In response to increasing environmental awareness, the use of biocontrol agents for plant disease suppression is emerging as a viable alternative. Biological control relies on the complex interactions (agonistic and antagonistic)—between plants and microbes living in the rhizosphere and phyllosphere. Various organisms including fungi, bacteria, mycorrhiza, yeast, virus, algae, cyanobacteria and so on, were identified with biological control properties and thus can be applied in plant disease management. Research on the selection, evaluation, characterization and application of these agents has significantly increased over the past few decades. However, despite extensive global research, the adoption of biopesticides by farmers remains limited. To promote the development and effective use of bioformulations, it is essential to optimize the activity of biocontrol agents, share knowledge on formulation processes, and simplify registration procedures. This paper discusses the advancements, challenges and perspectives related to the application of novel biocontrol agents in plant disease management.
Keywords: Biocontrol, Modes of action, Research and development, Control
INTRODUCTION
Plant diseases caused by infectious pathogens have significantly impacted human society and the natural environment, damaging food production, economic development, ecological resilience, and landscapes throughout human history. Plant diseases result from complex interactions among plants, pathogens, and the environment. Plant diseases can occur in the entire crop production chain and remain as one of the greatest threats to the sustainable development of society (Savary et al., 2019). According to the Food and Agriculture Organization (FAO), 14% of global crop production losses are caused by plant diseases each year, with fungal diseases accounting for 42% and bacteria for 27% (Roca-Couso et al., 2021). In the long history of agriculture, humans have developed a variety of approaches to manipulate the interaction to create a system in favor of the growth and development of host plants but suboptimum to the establishment, reproduction, and transmission of pathogens (Bisht et al., 2020). Depending on circumstances of crop, pathogen, geographic location, technology availability, regulation policy, and other factors, these control approaches can be agronomic (viz., crop diversification and field hygiene), regulative (viz., quarantine and eradication), genetic (viz., disease resistance and tolerance), physical (viz., soil solarization and flooding), chemical (viz., pesticides and host-immunity inducer) and can be used individually or in combination (integrated disease management, IDM) to suppress causal pathogen, promote host immunity, or change the biotic and abiotic environment where host–pathogen interaction occurs (He et al., 2021). Over the past 50 years, the most common method for disease control has been the extensive use of chemical pesticides (Peshin et al., 2009; Peshin and Zhang, 2014; Zhang et al., 2011; Zhang, 2018). Chemical and synthetic pesticides are used in excessive amounts to control pests in crop fields and such excessive applications deteriorate soil fertility and ecosystem health (Zhang et al., 2011; Zhang, 2018). Concerns about the safety of chemical residues and possible emergence of pesticide resistance in some pest species have necessitated the development of substitutes for these synthetic agro-inputs. However, the high effectiveness and ease of utilization of these chemicals resulted in environmental contamination and the presence of pesticide residues in food, in addition to various social and economic problems. Today, in the light of growing concerns towards environmental safety, suppression of plant diseases through biocontrol agents (BCAs) is gaining ground as an alternative to traditional disease management strategies. Now it is essential to focus on the challenges involved in testing, formulating, and delivering newer potential biocontrol agents within the context of integrated disease management. The objective of this paper is to build-on recent detailed reviews on advances, challenges and perspectives in the application of novel biocontrol agents for plant disease management.
BIOCONTROL AGENTS
Organisms that originate from nature and are utilized to control pests, weeds and diseases are known as biocontrol agents. Biocontrol agents can be classified into four distinct types: i) Macrobials, which encompass insects, mites, and beneficial nematodes that target pests; ii) Microbials, which are microorganisms like bacteria, fungi, and viruses, along with their derivatives. Microbial products may include these microorganisms, their metabolites, or fragments of their cells, and they can combat pests through direct infection, competitive displacement, or by forming a physical barrier; iii) Semiochemicals, chemical compounds emitted by plants and animals that send signals capable of influencing pest behavior. These are employed as repellents, attractants, or to disrupt mating; iv) Natural substances sourced from plants, minerals, or animals, or artificially created to replicate these natural compounds. They serve to repel and control both microbes and insects (Kumar et al., 2019). Additionally, it encompasses modified organisms, genes or a gene product used to suppress the growth of the unwanted organism (pest/pathogen) and to enhance the growth and yield of the wanted organism (crops, microorganism, or insects). The use of biocontrol agents to control plant diseases and pests is called biocontrol. It is a method of plant disease management by inhibiting plant pathogens, improving plant immunity, and/or modifying the environment through the effects of beneficial microorganisms, compounds, or healthy cropping systems (Burketova et al., 2015). Biological control offers several advantages over other approaches of plant disease management (Figure 1).
MECHANISMS EMPLOYED BY BIOCONTROL AGENTS FOR MANAGEMENT OF PLANT DISEASES
Biological control agents protect crops from diseases caused by plant pathogens via different modes of action. The biocontrol activity is exerted either directly through antagonism of plant pathogens or indirectly by eliciting a plant-mediated resistance response. Direct antagonism results from physical contact and/or a high degree of selectivity for the pathogen, by different mechanism(s) expressed by the biocontrol agents. Indirect antagonism results from activities that do not involve sensing or targeting a pathogen by the biocontrol agent/s (Figure 2). Stimulation of plant host defence pathways by biocontrol agents is the most indirect form of antagonism (Köhl et al., 2019; Hegde et al., 2022).
Biological control is dependent on numerous agonistic and antagonistic interconnections between plants and microbes living in the rhizosphere and phyllosphere (Mishra et al., 2015) and their application to minimize disease and subdue pests. Organisms from the rhizosphere can be harnessed from the surrounding environment (the black box approach) or can be introduced into the field from external sources (the silver bullet approach). It is beneficial to apply a consortium of microbes with collaborative properties rather than relying on a single organism since microbial consortia make up a stable rhizosphere that offers more effective control against pathogens (Ram et al., 2018). Apart from microbial applications, the utilization of other plant products like extracts, biofertilizers, and biopesticides, natural enemies of pests and pathogens, and gene products also aid in carrying out biological control (Ab et al., 2018).
ADVANCES IN RESEARCH AND DEVELOPMENT OF NOVEL BIOCONTROL AGENTS IN MANAGEMENT OF PLANT DISEASES
There is an increasing preference for food produced in ways that impart as little environmental impact as possible. Biocontrol agents provide a natural and environmentally acceptable alternative to conventional pesticides, therefore lowering agriculture’s ecological imprint. Biocontrol agents play an important part in sustainable agriculture by promoting natural pest control processes and lowering the need for synthetic pesticides. Government rules and incentives aimed at improving sustainable agriculture increase the market for biocontrol chemicals. Therefore, the transition to more environment-friendly pest management corresponds with the usage of biocontrol agents, boosting market expansion as farmers seek effective and ecologically favorable solutions. Furthermore, customers’ growing desire for organic products fuels this trend, forcing farmers to invest in biocontrol methods to fulfil market needs while remaining organically certified. According to research, global biocontrol agent’s market was valued at US$5.8 billion in 2023 and is expected to reach US$10.6 billion by 2032, at a CAGR of 8.1% during the forecast period. Based on formulation, the liquid formulation segment dominated the market in 2022 with a market share of 40% and is expected to keep its dominance during the 2024-2032 forecast period. Based on the application method, the foliar spray segment dominated the market in 2022 with a market share of 55% and is expected to keep its dominance during the 2024-2032 forecast period. By region, Biocontrol agent’s market is segmented into North America, Europe, Asia-Pacific, Latin America, the Middle East & Africa. The Asia-Pacific dominated the global biocontrol agents market in 2022 with a market share of 45% and is expected to keep its dominance during the forecast 2024-2032 period (www.custommarketinsight.com). Overall, biocontrol agent’s registrations are increasing globally, the expansion of various technologies has increased the scope for more products and the change in the trend to develop biocontrol agents is definitely on the rise (Figure 3).
A group of novel biocontrol agents that effectively control diseases in economically important crop species are discussed (Table 1).
Table 1. Groups of successful biocontrol agents that effectively control diseases in economically important crop species
Groups
Biocontrol agent
Target pathogens
Crops
Diseases
References
Fungi
T. harzianum
Crinipellis perniciosa
Cocoa
Witches’ broom
Marco et al. (2003)
T. lignorum
R. solani
Bean
Damping off
Aziz et al. (1997)
T. viride,
T. pseudokoningii,
T. koningii
Sclerotium cepivorum
Onion
White rot
Clarkson et al. (2002)
T. harzianum
Penicillium expansum
Apple
Blue and grey mould
Batta (2004)
T. virens, T. harzianum
R. solani
Potato
Stem canker or black scurf
Brewer and Larkin (2005)
T. koningii, T. aureoviride,
T. longibrachiatum
Sclerotinia sclerotiorum
Sunflower
Head rot
Escande et al. (2002)
T. asperellum
F. oxysporum
Tomato
Wilt
Cotxarrera et al. (2002)
T. koningii
Gaeumannomyces graminis var. tritici
Wheat
Take-all disease
Simon (1989)
T. virens
Verticillium dahliae
Cotton
Wilt
Hanson (2000)
T. afroharzianum
Uncinula necator
Grapes
Powdery mildew
Saxena et al. (2021)
Purpureocillium lilacinum (=Paecilomyces lilacinus) IIHR- Pl-2 (NAIMCC-SF-0034/ITCC 6887)
Meloidogyne incognita
Brinjal, tomato, carrot and okra
Root knot nematode
Saxena et al. (2021)
Pochonia chlamydosporia (=Verticillium chlamydosporium) IIHR- Vc-3(NAIMCC-SF-0035/ITCC 6898)
Meloidogyne incognita
Brinjal, tomato, carrot and okra
Root knot nematode
Saxena et al. (2021)
Trichoderma viride + Trichoderma harzianum
Pythium, Rhizoctonia,
Phytophthora and Fusarium
Many hosts
–
Saxena et al. (2021)
Pochonia chlamydosporia
Fusarium oxysporum f.sp. ciceris and F. udum, Meloidogyne incognita
Chickpea and redgram
Wilt disease complex
Saxena et al. (2021)
Gliocladium. spp.
Fusarium, Rhizoctonia,
Sclerotium, and Pythium
Many hosts
Soil borne diseases
Saxena et al. (2021)
Trichoderma reesei CSR-T-3 (NAIMCC-SF-0030)
Fusarium oxysporum f. sp. cubense Tropical race 4 and race 1
Banana
Fusarium wilt
Saxena et al. (2021)
Aspergillus niger AN-27
Pythium, Fusarium,
Macrophomina,
Rhizoctonia and Sclerotinia
–
–
Prashant et al. (2016)
Trichoderma asperelloides 5R (NAIMCC-SF-0026)
Uncinula necator
Grapes
Powdery mildew
Saxena et al. (2021)
Trichoderma harzianum IARI P-4(MTCC 5371)
Fusarium oxysporum f. sp. ciceris, Sclerotium rolfsii, Sclerotinia sclerotiorum,
Rhizoctonia solani, R. bataticola, Fusarium
oxysporum f. sp. lycopersici, Pythium ultimum
Chickpea, tomato, mungbean French bean
Soil borne diseases
Saxena et al. (2021)
Trichoderma viride
Fusarium, R. solani,
Macrophomina phaseolina and Colletotrichum
Many hosts
Soil borne diseases
Prashant et al. (2016)
Trichoderma harzianum Th4d (NAIMCC-F-02188)
Phytophthora sp., Macrophomina phaseolina, Fusarium sp., Botrytis cinerea, Alternaria sp. and Golovinomyces cichoracearum
Safflower, castor, aster, sunflower
Seedling blight, root rot, wilt, grey mold, leaf blight, powdery mildew
Saxena et al. (2021)
Trichoderma erinaceum
Rhizoctonia solani, Sclerotium rolfsii, Helminthosporium oryzae and Sclerotium oryzae
Rice
Soil borne plant pathogens, brown leaf spot
Swain et al. (2018)
Bjerkandera adusta BK-1
–
Tomato
Grey mould, brown spot, and early blight
Chen et al. (2021)
Yeast groups
Cryptococcus albidus var. aerius, Pichia guilliermondii,
Debaryomyces hansenii
Botryodiplodia theobromae
Mango
Post-harvest rot
Sugiprihatini et al. (2011)
P. guilliermondii
Aspergillus flavus
Soybean
Post-harvest infection
Wisniewski et al. (1991)
P. membranefaciens, C. albidus
Monilinia fructicola, Penicillium
expansum, Rhizopus
stolonifer
Apple
Post-harvest
infection
Chan and Tian
(2005)
P. guilliermondii
P. digitatum
Citrus
Decay of citrus fruit
Droby et al. (1993)
C. laurentii
M. fructicola, P. expansum
Peach
–
Yao and Tian (2005)
Chaetomium globosum
Phoma betae, P. ultimum, R. solani
Sugar beet
Damping-off
Walther and
Gindrat (1988)
Oomycetes
Pythium oligandrum
Sclerotinia sclerotiorum
Wheat
White mould/stem rot
Madsen and de
Neergaard (1999)
Verticillium dahliae
Pepper
Wilt
Rekanovic et al. (2007)
Pythium dissotocum
Tomato
Root rot
Vallance et al. (2009)
P. parasitica
Tomato
Buckeye rot
Picard et al. (2000)
B. cinerea
Tomato
Grey mould
Lou et al. (2011)
R. solani
Potato
Black scurf
Ikeda et al. (2012)
Aphanomyces cochlioides
Sugar beet
Root rot
Takenaka and
Ishikawa (2013)
Cercospora beticola
Sugar beet
Leaf spot
Takenaka and
Tamagake (2009)
Phytoplasma
Tobacco
–
Lherminier et al. (2003)
Mycorrhiza
Glomus mosseae
Thielaviopsis basicola
Tobacco
Root infection
Baltruschat and
Schoenbeck (1975)
Vesicular arbuscular mycorrhiza
R. solani
Rape seed
Damping-off
Iqbal et al. (1977)
Glomus fasciculatus
S. rolfsii
Peanut
Southern blight
Krishna and
Bagyaraj (1983)
Bacteria
Pseudomonas chlororaphis
F. oxysporum f. sp. radicislycopersici
Tomato
Foot and root
rot
Bolwerk et al. (2003)
P. fluorescens
Phytophthora infestans
Potato
Late blight
Hunziker et al. (2015)
Sarocladium oryzae
Rice
Sheath rot
Saravanakumar et al. (2009)
Banana bunchy top virus
Banana
Bunchy top disease
Kavino et al. (2008)
Dematophora necatrix
Avocado
Root rot
Cazorla et al. (2006)
Pseudomonas fluorescens IIHR Pf-2 (NAIMCC-SB-0038/ITCC B0034) and Trichoderma harzianum IIHR Th-2 (NAIMCC-SF-0033/ITCC 6888)
Meloidogyne incognita, Ralstonia solanacearum, Erwinia carotovora, Fusarium oxysporum f. sp. vasinfectum, Fusarium solani
Tomato, cotton
Root knot nematode, bacterial wilt, vascular wilts
Saxena et al. (2021)
Pseudomonas fluorescens
(NAIMCC-SB-0053)
–
Wheat, rice tomato, chickpea
Spot blotch, sheath blight and wilt
Saxena et al. (2021)
P. putida
Fusarium sp.
Radish
Wilt
de Boer et al. (2003)
Bacillus pumilus, B. amyloliquefaciens, B. subtilis
Cucumber mosaic virus
Tomato
Mosaic disease
Zehnder et al. (2000)
B. amyloliquefaciens, B. subtilis, B. pumilus
Tomato mottle virus
Tomato
Tomato mottle
Murphy et al. (2000)
B. pumilus
Erwinia tracheiphila
Cucumber
Bacterial wilt
Zehnder et al. (2001)
Peronospora tabacina
Tobacco
Blue mould
Zhang et al. (2002)
B. subtilis, B. pumilus
Sclerospora graminicola
Pearl millet
Downy mildew
Niranjan Raj et al. (2003)
B. cereus
Corynespora cassiicola
Tomato
Foliar diseases
Silva et al. (2004)
Bacillus sp.
Phytophthora capsici
Bell pepper
Blight
Jiang et al. (2006)
Magnaporthe grisea
Rice
Blast
Naureen et al. (2009)
P. capsici
Squash
Blight
Zhang et al. (2010)
B. amyloliquefaciens
F. oxysporum
Maize
Wilt
Koumoutsi et al. (2004)
Brevibacillus brevis
B. cinerea
Cucumber
Grey mould
Konstantinidou-Doltsinis et al. (2002)
Enterobacter agglomerans
R. solani
Cotton
Root rot
Chernin et al. (1995, 1997)
Paenibacillus sp.
F. oxysporum
Sorghum
Wilt
Budi et al. (2000)
Lysobacter enzymogenes
F. graminearum
Wheat
Wilt
Li et al. (2008)
Stenotrophomonas maltophilia
P. ultimum
Sugar beet
Damping-off
Dunne et al. (2000)
Agrobacterium radiobacter
Agrobacterium tumefaciens
Many hosts
–
Vicedo et al. (1993)
Collimonas fungivorans
F. oxysporum
Tomato
Wilt
Kamilova et al. (2007)
L. enzymogenes
Pythium aphanidermatum
Cucumber
Root and crown rot
Folman et al. (2003)
Burkholderia cepacia
Pythium sp. and
Aphanomyces sp.
Peas
Damping off,
root rot
Heungens and
Parke (2000)
Bacillus velezensis K01
Botrytis cinerea
Tomato and pepper
–
Xue et al. (2023)
Actinobacteria
S. janthimts,
S. cinerochromogenes
Pythium coloration
Carrot
Cavity spot
El-Tarabily et al. (1997)
Streptomyces species
Phytophthora medicaginis and Phytophthora sojae
Alfalfa and soybean
Root rot
Xiao et al. (2002)
Plectosporium tabacinum
Lupin
Root rot
El-Tarabily (2003)
Pythium aphanidermatum
Cucumber
Damping-off
El-Tarabily (2006)
Actinomadura sp.
Phytophthora cinnamomi
Snapdragon
Root rot
You et al. (1996)
Micromonospora sp.
Gaeumannomyces graminis var. tritici
Wheat
Take all
Coombs et al. (2004)
Fusarium oxysporum f. sp. lycopersici
Tomato
Wilt
Smith (1957)
M. carbonacea
P. coloratum
Carrots
Cavity spot
El-Tarabily et al. (1997)
Sclerotinia minor
Lettuce
Basal drop
El-Tarabily et al. (2000)
M. chalcea
P. aphanidermatum
Cucumber
Damping-off
El-Tarabily (2006)
Microbispora rosea
P. aphanidermatum
Cucumber
Damping-off
El-Tarabily (2006)
G. graminis var. tritici
Wheat
Take all
Coombs et al. (2004)
Streptosporangium albidum,
Streptoverticillium netropsis
P. coloratum
Carrots
Cavity spot
El-Tarabily et al. (1997)
Arthrobacter sp.
Fusarium moniliforme var. subglutinans
Pine
Pitch canker
Barrows-Broaddus and
Kerr (1981)
Virus
Zucchini yellow mosaic virus (mild strain)
Severe strains of same
Cucurbits
Mosaic
Lecoq and Lemaire (1991)
Papaya ringspot virus (mild strain)
Severe strains of same
Papaya
Ring spot disease
Tennant et al. (1994)
Mild strain of Citrus tristeza virus (mild strain)
Severe strains of same
Citrus
Tristeza
Folimonova (2013)
Fungal agents for biocontrol
The well-known fungal biocontrol agents include the Trichoderma species, ectomycorrhizas, arbuscular mycorrhizas (AMF), yeasts and endophytes. Even the nonvirulent strains of certain pathogens can utilize hypovirulence-associated mycoviruses in order to function as biocontrol fungi (Ghorbanpour et al., 2018). There are 300 fungal antagonists belonging to 13 classes and 113 genera that were used against fungal plant pathogens. Trichoderma is identified as the genus (comprising 25 species) with greatest potential of biocontrol and is used as a biocontrol agent against a number of plant fungal diseases. Trichoderma species namely T. atroviride, T. hamatum, T. harzianum and T. viride are effective in the control of soil borne fungal pathogens (Hegde and Vijaykumar, 2023). In addition, some more antagonistic species, Alternaria, Aspergillus, Candida, Fusarium, Gliocladium, Penicillium, Pichia, Pythium, Talaromyces and Verticillium play important roles in the management of plant diseases (Hegde and Jahagirdar, 2020; Kasun et al., 2020). Microbial communities can inhabit both external surfaces (epiphytes) or internal plant tissues (endophytes), and these communities play an important role in protecting plants from diseases (Parasuraman et al., 2019). Numerous metabolites with various chemical structures, including terpenoids, alkaloids, steroids, peptides, isocoumarins, benzopyranones, and quinones, have been identified in endophytic fungi. The identification of these metabolites provided a solid chemical basis for the development of agrochemicals that may have antibacterial, antifungal, herbicidal, nematicidal, insecticidal and other agricultural uses (Risoli et al., 2022). Biocontrol agents Chaetomium sp. and Athelia bombacina were successful in suppression of Venturia inaequalis; Rhodotorula kratochvilovae strain LS11 controlled Monilinia spp., while Tuberculina maxima parasitizes the white pine blister rust fungus Cronartium ribicola; Darluca filum and Verticillium lecanii parasitize several rusts, Tilletiopsis sp. parasitizes the cucumber powdery mildew fungus Sphaerotheca fuliginea, and Nectria inventa and Gonatobotrys simplex parasitize two pathogenic species of Alternaria (Agrios, 2005). A novel isolate, Trichoderma erinaceum isolated from above-ground habitats effectively controlled three soil borne plant pathogens of rice i.e., Rhizoctonia solani, Sclerotium rolfsii and Sclerotium oryzae under controlled conditions and R. solani and Helminthosporium oryzae under field conditions while improving the germination rate and vigor of rice plant (Swain et al., 2018). The fungal strain, Bjerkandera adusta BK-1, is a novel promising broad-spectrum biocontrol agent against multiple plant fungal diseases (Chen et al., 2021).
Mycorrhizal fungi as biocontrol agents
Several studies lay emphasis on the biocontrol abilities of Arbuscular mycorrhizal fungi (AMF) as they have been shown to reduce the incidence of fungal diseases and nematode attacks on host plants by 30 to 42% and 44–57%, respectively (Veresoglou and Rillig, 2012; Mishra et al., 2018). AMF offers defence against several fungal pathogens belonging to the genera Colletotrichum, Alternaria, Erysiphe, Gaeumannomyces, Macrophomina, Botrytis, Rhizoctonia, Fusarium, Cylindrocladium, Sclerotium and Verticillium (Singh, 2017). Notable ectomycorrhizal species such as Amanita spp., Boletinus spp., Cenococcum spp., Lactarius spp., Laccaria laccata, Pisolithus tinctorius, Suillus luteus and Thelephora terrestris are known to generate metabolites that safeguard plant root systems from diseases (Trappe, 1962). Suillus luteus has been identified as an effective bio control agent against damping-off disease caused by Fusarium verticillioides and Fusarium oxysporum in Scots and Stone pine trees (Mateos et al. 2017).
Biocontrol by yeasts
Yeasts such as Aureobasidium pullulans, Cryptococcus albidus, Candida oleophila, Saccharomyces cerevisiae, and Metschnikowia fructicola are currently being employed as biocontrol agents as they are effective adversaries of various plant pathogens (Pandit et al. 2022). Biofungicides based on Aureobasidium pullulans strains indicated for biocontrol applications against fungal pathogens of fruit (e.g., Boni protect®) are available in the market (Weiss et al., 2006). The BCA Papiliotrema terrestris strain LS28 was isolated from apple epiphytic microflora and selected for its ability to counteract fungal pathogens of plants and fruits, both in the field and in postharvest stages (Palmieri et al., 2021). Low temperature-adapted yeasts Leucosporidium scottii and Cryptococcus laurentii were highly effective in their biocontrol activity on apple and tomatoes inoculated with Botrytis cinerea in cold storage conditions (Hu et al., 2017).
Bacteria for biocontrol
A wide variety of bacterial genera, including Agrobacterium, Alcaligenes, Arthrobacter, Bacillus, Enterobacter, Erwinia, Pseudomonas, Rhizobium, Serratia, Stenotrophomonas, Streptomyces, Xanthomonas have been described to have plant disease protection activity against fungal and bacterial pathogens (Bonaterra et al., 2022). The activity of P. fluorescens EPS62e and P. pseudoalcaligenes AVO110 in the reduction of Erwinia amylovora or Rosellinia necatrix infections, respectively, is based on their strong fitness in colonizing plant tissues as they have higher growth potential and nutrient use efficiency than the target pathogens (Cabrefiga et al., 2007; Pliego et al., 2008, Dobhal and Hegde, 2021). In Vitis, P. fluorescens PTA-CT2 induces ISR to Plasmopara viticola and Botrytis cinerea that depends on the activation of SA or JA and ABA defensive pathways (Lakkis et al., 2019). Species of Bacillus including B. subtilis, B. amyloliquefaciens, B. methylotrophicus, B. polymyxa, B. cereus, B. coagulans, B. subterraneous, B. licheniformis, B. pumilus, B. circulans, B. velezensis, B. megaterium, B. firmus, B. aquimaris, B. vietnamensis, and B. aerophilus have been reported to be suitable for biological control of plant disease and plant growth promotion (Fan et al., 2017; Saxena et al., 2020; Hegde and Malligawad, 2020). Bacillus spp. produce several bacteriocins with antimicrobial activity such as amylolysin, amylocyclicin, amysin, subtilin, subtilosin A, subtilosin B, thuricin. Bac-GM17 produced by B. clausii GM17 have activity against Agrobacterium tumefaciens (Mouloud et al., 2013) or thuricin Bn1 from B. thuringiensis subsp. kurstaki Bn1 against Pseudomonas savastanoi and Pseudomonas syringae (Ugras et al., 2013). B. amyloliquefaciens FZB42 produced secondary metabolites (surfactin, fengycin, and bacillomycin D) that trigger plant defense gene expression and contribute to lettuce bottom rot reduction (Chowdhury et al., 2015). A novel biocontrol agent Bacillus velezensis K01 has a broad-spectrum antagonistic activity and demonstrated a biocontrol efficiency of over 78% against preharvest and postharvest grey mold diseases in tomato and pepper (Xue et al., 2023). Streptomyces CBQ-EA-2 and CBQ-B-8 have chitinolytic, cellulolytic, and proteolytic activity and result in reduced Macrophomina phaseolina and Rhizoctonia solani infections in Phaseolus vulgaris (Díaz-Díaz et al., 2022). Pantoea species were shown to target a wide spectrum of plant pathogens including bacteria, fungi, and oomycetes via secretion of antimicrobial compounds such as pantocins, herbicolins, microcins, and phenazines (Walterson and Stavrinides, 2015). Lactobacillus plantarum PM411 and TC92 are effective in preventing bacterial plant diseases (Daranas et al., 2019).
Plant viruses and their biocontrol nature
Viruses are not regarded as living organisms, but certainly as valid agents of biological control, and hence they are included (with these caveats) within our definition of living agents (Stenberg et al., 2021). Viruses have been increasingly used to combat pests both directly (by pathogenic pest infection) and indirectly (by cross-protection, i.e., ‘vaccinating’ crops with mild virus strains), and placed in the canon of biological control with no conceptual opposition from the scientific community (Di Giallonardo and Holmes, 2015). Qu et al. (2021) have elucidated the effects of a single-stranded DNA virus, Sclerotinia sclerotiorum hypovirulence-associated DNA virus 1 (SsHADV-1) that infects the fungus Sclerotinia sclerotiorum, a disease causative agent of many crops and they have further elucidated the altered expression of phenotype related genes upon SsHADV-1 infection by using digital RNA sequencing.
Phage-based biocontrol
Phages have been in use as biocontrol agents against bacterial pathogens for a long time. Peptidoglycan hydrolases, lysins from phages Atu_ph02 and Atu_ph03 are capable of blocking cell division in Agrobacterium tumefaciens (causes crown gall disease) resulting in its lysis (Attai et al., 2017). Other lysins from CMP1 and CN77 phages have also shown lytic capacity against Clavibacter michiganensis subsp. michiganensis, that cause bacterial wilt and canker of tomato (Wittmann et al., 2016). Mostly, the application of phages and phage lysins in plant disease management is a progressive step and has shown positive outcomes in a number of instances. Focus now needs to be on developing better delivery methods and guaranteeing a longer shelf life for the phage and its enzymes on the host plant (Dy et al., 2018) to facilitate effective biocontrol.
Algal and cyanobacterial biocontrol activities
Algal and cyanobacterial extracts are a rich source of bioactive elicitors (Jimenez et al., 2011) with antifungal, antiviral and antibacterial properties (Arunkumar et al., 2010). Activity of polyphenol oxidase and peroxidase enzymes improved when extracts from Cystoseira myriophylloides, Laminaria digitata and Fucus spiralis were utilized against Verticillium dahliae wilt of tomato (Esserti et al., 2017). Employing Nostoc entophytum and Nostoc muscorum in the soil against Rhizoctonia solani greatly enhance seedling endurance along with improving root and shoot dry weight and plant length (Osman et al., 2011). Usage of Cyanobacteria, Anabaena sp. on zucchini cotyledons infected with powdery mildew (Podosphaera xanthii) resulted in enhanced enzymatic activity of peroxidases, endochitinase, chitin 1,4-β-chitotriosidase, β-N-acetylhexosaminidase, and β-1,3-glucanase (Roberti et al., 2015).
ADVANCED BIOCONTROL STRATEGIES
FOR THE MANAGEMENT OF PLANT DISEASES
Microbial volatilome and its role in the biological control
Among the several microbiological strategies used by BCAs, the production of volatile organic compounds (VOCs) is a method that is helpful in situations where the straightforward association between the pathogen and its competitor is not possible. All living forms synthesize VOCs, and these can be exploited for usage in biocontrol of plant pathogens like bacteria, oomycetes, and fungi. VOCs are a sustainable preference for synthetic fungicides due to their ease of application, low residue deposition in the environment and on crops, and their biocontrol efficacy (Tilocca et al., 2020). In vitro studies indicated that Bacillus volatile compounds reduced Ralstonia growth and viability and caused significant problems in cell integrity and motility in addition to considerable alterations in Ralstonia genes expression that controls tobacco wilt disease progression (Tahir et al., 2017). It’s possible that bacterial volatiles have a role in Bacillus reported biocontrol properties both directly and indirectly, and that bacterial VOC bouquets function as multifactorial, sequential, or simultaneous signals on pathogens and hosts (Bailly and Weisskopf, 2017).
Microbiome-based solutions for plant protection
A wide range of microorganisms effectively interact with plants in the rhizosphere region. Microbial invasion can affect the network of microorganisms that are linked with plants. These network models of soil and plant microbiomes can be interpreted for biocontrol and present new prospects for disease management. While single organisms were commonly utilized in the past, their effects were often uneven, microbiome-based biocontrol techniques are now possible (Berg et al., 2017; Jahagirdar et al., 2021). Microbial consortia offer numerous benefits for managing plant diseases compared to the application of a single strain such as i) Consortia of bio-control agents targets wide range of phytopathogens, ii) Enhancement of soil suppressiveness through increased microbial diversity within the soil rhizosphere, iii) Diverse species occupy different ecological niches in rhizosphere and thereby restricts competition between them, iv) Improved rhizosphere colonization due to interactions among microbes within the consortium, v) Utilization of multiple strains with varied modes of action, which boosts the bio-control efficiency of the consortia. Mixture of four bacterial isolates comprising of Serratia marcescens, Pseudomonas fluorescens, Rahnella aquatilis and Bacills amyloliquefaciens improved soil suppressiveness against Fusarium oxysporum f.sp. ciceris race 0 and Fusarium solani f.sp. pisi causing Fusarium decline in chickpea by increasing microbial diversity in rhizosphere (Palmieri et al., 2017). In the future, microbial consortia and biocontrol agents can be employed to improve biodiversity associated with crops via microbiome engineering to achieve definitive microbiome outcomes as desired (Erlacher et al., 2014; Jadav et al., 2022).
Microbiome engineering
Plant microbiome engineering can be achieved in two ways: bottoms-up methods involve isolating, altering, and reviving certain microbes, whereas top-down approaches involve synthetic ecology, which includes horizontal gene transfer to a variety of hosts in situ and then phenotyping the microbiome (Ab Rahman et al., 2018). The root microbiome can be engineered by soil conditioning techniques such as incorporating legumes to enrich native diazotrophic microorganisms. Additionally, the use of substrates/non-microbial biostimulants, including innovative organic soil amendments and root exudates, can help attract beneficial microbiota. Introducing external microbial inoculations, whether through soil application or as endophytes, can effectively manage Xanthomonas blight in rice (Saikia et al. 2020). Another strategy is to alter indigenous microbial strains through genetic engineering techniques, including CRISPR/Cas9 and RNA interference. This modification seeks to enhance the microbes' ability to withstand stress and optimize their nutrient utilization (Joshi et al. 2025). It would be extremely helpful to develop microbiomes that are long-lasting, stress-resistant, and capable of increasing agricultural output. Microbiome bioengineering is a fascinating choice for improving plant health and will provide enormous benefits to biocontrol methods in the future (Orozco-Mosqueda et al., 2018). Plant microbiome bioengineering is an intriguing option for improving a plant’s biological capabilities, an approach that, while still in its infancy, has the potential to be of immense agricultural value (del Carmen et al., 2018).
Genetically modified biocontrol agents
The recent advancements in genome engineering tools, genome-wide functional tools and meta-omic tools can improve our ability to design microbes for biocontrol and biofertilization, as well as increase agricultural productivity and yield. Rhizoctonia solani infection in beans can be brought under control by transferring a gene coding for the enzyme chitinase from Serratia to a Pseudomonas endophyte (Downing and Thomson, 2000). Clermont et al. (2011) employed genome shuffling to create superior Streptomyces melanosporofaciens EF76 biocontrol strains. Four strains with improved antagonistic activity against the potato diseases Streptomyces scabies and Phytophthora infestans were isolated after two rounds of genome shuffling. Biological control ability can also be improved by employing chemical mutagenesis. Examples include the use of nitrosoguanidine mutagenesis in Pseudomonas aurantiaca B-162 to produce a strain with better phenazine synthesis leading to improved biocontrol activity (Feklistova and Maksimova, 2008) and Trichoderma harzianum strains that exhibited enhanced biocontrol ability after UV mutagenesis (Marzano et al., 2013). A recombinant strain of Pseudomonas synxantha, designated ZHW 15 and ZHW 25, demonstrated enhanced biocontrol efficacy against soil-borne pathogens affecting wheat (Zhang et al. 2020). The addition of the required mutation can at times produce altered gene expression in non-targeted genes resulting in undesired effects. These constraints can be overcome using more recently established genome editing approaches. We can insert mutations into specific regions in the genome with high precision and efficiency using techniques like Crispr/Cas (Barrangou and van Pijkeren, 2016). The gene editing approach could also help in commercialization of BCA’s through ease of regulatory clearances.
Mycoviruses as biocontrol agents
Mycoviruses having capability to infect fungal pathogens are known to have the potential to be used as biological control agents against plant diseases. The mycoviruses were recognized to induce hypovirulence (reduced virulence) in their hosts and this notion elicited great interest in characterization of viruses from phytopathogenic fungi and to utilize them as biocontrol agents (García-Pedrajas et al., 2019). Surprisingly, numerous plant pathogenic fungi are found to harbor mycoviruses, which reduce the virulence of their fungal host (Kumar and Chandel, 2016). Hypovirulence-associated mycoviruses are those mycoviruses that reduce the pathogenicity of their inhabiting fungal hosts. However, it is difficult to transmit these mycoviruses easily between vegetatively incompatible groups and hence it has been difficult to develop commercial mycovirus biocontrol strategies for phytopathogenic fungi (Khalifa and MacDiarmid, 2021).
MAJOR CHALLENGES FOR BIOCONTROL RESEARCH IN THE PLANT DISEASE MANAGEMENT
Biological control strategy was initially employed at the end of the 19th century. Displaying an array of advantages, the literature has also mentioned many limitations of this biocontrol strategy for controlling plant diseases (Stenberg et al., 2021). Though these methods offer effective alternatives to chemical pesticides for controlling pests and plant diseases, there are still various challenges to overcome. These challenges are briefly discussed in the subsequent paragraphs.
The journey of biocontrol agents from lab to field
Researchers are screening potential microbes as part of their work to create biological pesticide remedies that are very effective for controlling plant diseases in agriculture. These strains are usually chosen based on factors such as disease resistance, host range, availability, formulation, mass production, and farmer practice (Farrar et al., 2014). Bioagents can be assessed by considering geographical locations, host crop species, soil types, and environmental factors. The growth of BCAs is typically easier to observe in controlled environments such as greenhouses. The preference of most researchers at this stage might be associated with the reliability of the environment (Spadaro and Gullino, 2004). The performance of BCAs in greenhouse trials can provide important theoretical and practical support for field utilization. The stability of BCAs is affected by the method of formulation, shipment, and storage environment. To achieve high levels of BCA strategy success, it is important to improve the formulation technology, extend the storage duration of the BCA product, optimize the production of selected microbial strains, and achieve large-scale application through low-cost manufacturing companies (Barratt et al., 2018). During the formulation and field efficacy trials, private sector partners and licensed laboratories conduct the essential environmental and human health assessments, as well as quality assurance for the ultimate commercialization of biopesticides. Before utilizing biopesticides on a large scale in the field, government authorities must grant permission. Typically, the biopesticide registration and regulation portfolio includes modified versions of synthetic pesticides as well as risk evaluation. This includes ecotoxicological and toxicological tests, as well as studies into their mechanism of action and host spectrum. Most of these requirements are difficult for authorities to achieve because producing effective bioagents while maintaining acceptable safety and consistency standards for commercialization might be a difficult task (Chandler et al., 2011).
Limited number of registered bioagents and lack of awareness
Despite their well-documented efficacy, BCAs now account for less than 5% of the crop protection sector’s commercial value (He et al., 2021). The identification, characterization, and registration of potential microbes takes more time and requires academic-industry collaborations (Stenberg et al., 2021). Furthermore, using natural resources to manage diseases (i.e., BCAs) raises several ethical and legal issues that might affect the biodiversity of an area (Sundh and Eilenberg, 2020). In this situation, new BCA species and populations have been restricted from entering specified countries. At present, every country has its own regulatory system, which varies widely. For example, high development costs for new commercial BCAs have been recognized as an obstacle to the BCA industry’s development in Australia (Hidayah, 2022). The standards for data submission for temporary registration in India are less demanding than those for standard registration. It is imperative to meet the quality standards set forth by the Central Insecticides Board, which encompass content, the virulence of the organism in terms of LC50, moisture content, shelf life, and secondary non-pathogenic microbial load. Biopesticides are exempted from the fees for pesticide registration and for the right to sell the product in Canada, except for the cost charged for reviewing the product label. Like Canada, the US does not require registration of indigenous beneficial insects or entomopathogenic nematodes or similar types of microbial inoculants as long as they do not make a pest control claim. In the U.S. and EU, biopesticide registration can take over 3 years as compared to 6-12 months for synthetic products. The timeline for obtaining approval and registration of a microbial pest control product, starting from the pre-consultation with the Pest Control Products Board, Kenya is influenced by the complexity of the product and generally ranges from 2 to 4 years (Hoeschle-Zeledon et al., 2013). To facilitate the registration and commercialization of novel BCAs and their products, BCA registration requires extensive cooperation among governmental institutes, universities, and industry sectors. The number of programs that provide financial and ecological benefits plays a vital role in bioagents registration (Stenberg et al., 2021). Hence, local usage and global marketing commercialization must fulfil international legislation. The International Biological Control Organization (IOBC) was established to bring together scholars, scientists, and professionals from a wide range of industries and fields to identify barriers and offer recommendations to overcome those (Brodeur et al., 2018). Biocontrol management has a direct impact on farmers’ expenses and income, but it also has an indirect impact on their financial benefits due to its effect on farmland biodiversity and environmental sustainability (Barratt et al., 2018). However, farmers have not fully utilized commercial bioagents due to a lack of information and awareness. Therefore, there is a need to strengthen the idea of biological management in the farming community to restore their trust in the use of bioagents (Ayaz et al., 2023; Hegde et al., 2023).
Bioagents commercialization and legislative procedure
Despite their growing popularity in crop disease management, biocontrol agents currently represent only 1% of agricultural control techniques, whereas synthetic-based pesticides account for 15% in the agriculture sector (Fravel, 2005). The commercialization of bioagents is a multi-step procedure that faces numerous challenges. Before being licensed for commercialization, similar to synthetic pesticides, BCAs are subjected to risk evaluations (Wilson and Jackson, 2013). The current trend of reducing the use of synthetic pesticides and simplifying the regulatory process for low-risk products may allow BCAs to be commercialized worldwide (Chandler et al., 2011; Hegde and Vijaykumar, 2022).
CONCLUSION AND PERSPECTIVES
Synthetic pesticides, due to their eco-toxicological risks, are facing increasing restrictions on their use worldwide. Biocontrol products based on microbial antagonists offer safer alternatives to replace or complement chemical pesticides. As a result, research on the selection, characterization and commercial development of BCAs is steadily increasing over the last few decades. Among the most studied biocontrol agents are fungi, bacteria and yeasts. Despite the large number of studies on biocontrol agents, the commercial formulations of BCA are still too limited and insufficient to meet the growing demand for sustainable agricultural systems. The main challenges hindering the development of new microbe-based formulation are (i) the sometimes lower efficacy of BCAs compared to synthetic pesticides, (ii) the lack of information available on the microbial formulation protocols, due to industrial secrecy and (iii) the complex registration and patent procedures in various countries. The use of molecular and omics tools could significantly improve the selection of biocontrol agents by providing a deeper understanding of their mechanisms of action, which is critical for optimizing BCAs activity and streamlining the registration processes. Future research should also investigate the economic feasibility and societal impact of expanding the use of biocontrol technologies. Analyzing the cost effectiveness and potential benefits for farmers and the agriculture sector would be valuable. For the widespread adoption of biocontrol strategies, it is essential to enhance dissemination among farmers, extension services, and policymakers. Efforts should focus on developing educational programs and outreach initiatives to raise awareness and make biocontrol solutions more accessible. Despite the technical and bureaucratic challenges associated with developing microbial BCAs, there is a growing shift towards plant disease control methods with lower environmental impact and fewer risks for human health. Additionally, political support for research into new technologies, particularly those addressing climate change and biodiversity conservation, is increasing. Consequently, research into the design and development of more efficient bioproducts, including microbial formulations to be used against plant diseases, will attract more and more attention in the near future.
REFERENCES
Ab Rahman, S. S. F., E. Singh, C. M. J. Pieterse and P. M. Schenk. 2018. Emerging microbial biocontrol strategies for plant pathogens. Plant Sci. 267: 102–111.
Agrios, G. N. 2005. Control of Plant Diseases. Academic Press: San Diego, CA, USA, pp. 293–353.
Arunkumar, K., S. R. Sivakumar and R. Rengasamy. 2010. Review on bioactive potential in seaweeds marine macroalgae: A special emphasis on bioactivity of seaweeds against plant pathogens. Asian J. Plant Sci. 9: 227–240.
Attai, H., J. Rimbey, G. P. Smith and P. J. B. Brown. 2017. Expression of a peptidoglycan hydrolase from lytic bacteriophages. Appl. Environ. Microbiol. 83: 14–17.
Ayaz, M., C. H. Li, Q. Ali, W. Zhao, Y. K. Chi, M. Shafiq, F. Ali, X. Y. Yu, Q. Yu, J. T. Zhao, et al. 2023. Bacterial and fungal biocontrol agents for plant disease protection: Journey from lab to field, current status, challenges, and global perspectives. Molecules. 28: 6735. https://doi.org/10.3390/molecules28186735.
Aziz, N. H., M. Z. El-Fouly, A. A. El-Essawy, M. A. Khalaf. 1997. Influence of bean seedling root exudates on the rhizosphere colonization by Trichoderma lignorum for the control of Rhizoctonia solani. Bot. Bull. Acad. Sin. 38: 33–39.
Bailly, A. and L. Weisskopf. 2017. Mining the volatilomes of plant-associated microbiota for new biocontrol solutions. Front. Microbiol. 8: 1638.
Baltruschat, H. and F. Schoenbeck. 1975. The influence of endotrophic mycorrhiza on the infestation on tobacco by Thielaviopsis basicola. Phytopath. Z 84: 172–188.
Barrangou, R. and J. P. van Pijkeren. 2016. Exploiting CRISPR-Cas immune systems for genome editing in bacteria. Curr. Opin. Biotechnol. 37: 61–68.
Barratt, B. I. P., V. C. Moran, F. Bigler and J. C. van Lenteren. 2018. The status of biological control and recommendations for improving uptake for the future. Bio Control. 63: 155–167.
Barrows-Broaddus, J. and T. J. Kerr. 1981. Inhibition of Fusarium moniliforme var. subglutinans, the causal agent of pine pitch canker, by the soil bacterium Arthrobacter sp. Can. J. Microbiol. 27: 20–27.
Batta, Y. A. 2004. Effect of treatment with Trichoderma harzianum Rifai formulated in invert emulsion on postharvest decay of apple blue mold. Int. J. Food Microbiol. 96: 281–288.
Berg, G., M. Köberl, D. Rybakova, H. Müller, R. Grosch and K. Smalla. 2017. Plant microbial diversity is suggested as the key to future biocontrol and health trends. FEMS Microbiol. Ecol. 93: 5.
Bisht, N., S. K. Mishra and P. S. Chauhan. 2020. Bacillus amyloliquefaciens inoculation alters physiology of rice (Oryza sativa L. var. IR-36) through modulating carbohydrate metabolism to mitigate stress induced by nutrient starvation. J. Biol. Macromol. 143: 937–951.
Bolwerk, A., A. L. Lagopodi, A. H. M. Wijfjes, G. E. M. Lamers, T. F. C. Chin-A-Woeng, J. J. Lugtenberg Ben and G. V. Bloemberg. 2003. Interactions in the tomato rhizosphere of two Pseudomonas biocontrol strains with the phytopathogenic fungus Fusarium oxysporum f. Sp. radicis-lycopersici. Mol. Plant-Microbe Interact. 16: 983–993.
Bonaterra, A., E. Badosa, N. Daranas, J. Frances, G. Rosello and E. Montesinos. 2022. Bacteria as biological control agents of plant diseases. Microorganisms. 10: 1759. https://doi.org/10.3390/microorganisms10091759.
Bora, L.C., R. Chetia, S. Saikia, and K. P. Baruah. 2018. Biopesticides: their role in sustainable agriculture, production technology and entrepreneurship opportunities in Assam. Dept. of Plant Pathology Assam Agricultural University. Jorhat, Assam.
Brewer, M. T. and R. P. Larkin. 2005. Efficacy of several potential biocontrol organisms against Rhizoctonia solani on potato. Crop Prot. 24: 939–950.
Burketova, L., L. Trda, , P. G. Ott and O. Valentova. 2015. Bio-based resistance inducers for sustainable plant protection against pathogens. Biotechnol. Adv., 33: 994–1004.
Cabrefiga, J., A. Bonaterra. and E. Montesinos. 2007. Mechanisms of antagonism of Pseudomonas fluorescens EPS62e against Erwinia amylovora, the causal agent of fire blight. Int. Microbiol. 10: 123–132.
Cazorla, F. M., S. B. Duckett, E. T. Bergstreom, S. Noreen, R. Odijk, B. J. Lugtenberg, J. E. Thomas-Oates and G. V. Bloemberg. 2006. Biocontrol of avocado dematophora root rot by antagonistic Pseudomonas fluorescens PCL1606 correlates with the production of 2-hexyl 5-propyl resorcinol. Mol. Plant-Microbe Interact. 19: 418–428.
Chan, Z. and S. Tian. 2005. Interaction of antagonistic yeasts against postharvest pathogens of apple fruit and possible mode of action. Postharvest Biol. Technol. 36: 215–223.
Chandler, D., A. S. Bailey, G. M. Tatchell, G. Davidson, J. Greaves and W. P. Grant. 2011. The development, regulation and use of biopesticides for integrated pest management. Philos. Trans. R. Soc. Lond. B Biol. Sci. 366: 1987–1998.
Chen, Y., Yunbao Wan, Xuefei Jiang, Qian Liu, Tao Wei and Maolin Wang. 2021. A novel fungal strain, Bjerkandera adusta BK-1, as a promising broad-spectrum biological control agent against plant fungal diseases. https://doi.org/10.1080/09583157.2021.1992347
Chernin, L., Z. Ismailov, S. Haran and I. Chet. 1995. Chitinolytic Enterobacter agglomerans antagonistic to fungal plant pathogens. Appl. Environ. Microbiol. 61: 1720–1726.
Chernin, L. S., L. Dela Fuente, V. Sobolev, S. Haran, C. E. Vorgias, A. B. Oppenheim, I. Chet. 1997. Molecular cloning, structural analysis, and expression in Escherichia coli of a chitinase gene from Enterobacter agglomerans. Appl. Environ. Microbiol. 63: 834–839.
Chowdhury, S. P., J. Uhl, R. Grosch, S. Alqueres, S. Pittroff, K. Dietel, P. Schmitt-Kopplin, R. Borriss and A. Hartmann. 2015. Cyclic lipopeptides of Bacillus amyloliquefaciens subsp. plantarum colonizing the lettuce rhizosphere enhance plant defense responses toward the bottom rot pathogen Rhizoctonia solani. Mol. Plant Microbe Interact. 28: 984–995.
Clarkson J. P., T. Payne, A. Mead and J. M. Whipps. 2002. Selection of fungal biological control agents of Sclerotium cepivorum for control of white rot by sclerotial degradation in a UK soil. Plant Pathol. 51: 735–745.
Clermont, N., S. Lerat and C. Beaulieu. 2011. Genome shuffling enhances biocontrol abilities of Streptomyces strains against two potato pathogens. J. Appl. Microbiol. 111: 671–682.
Coombs, J. T., P. P. Michelsen and C. M. M Franco. 2004. Evaluation of endophytic actinobacteria as antagonists of Gaeumannomyces graminis var. tritici in wheat. Biol. Control. 29: 359–366.
Cotxarrera, L., M, I, Trillas-Gay, C, Steinberg and C. Alabouvette. 2002. Use of sewage sludge compost and Trichoderma asperellum isolates to suppress Fusarium wilt of tomato. Soil Biol. Biochem. 34: 467–476.
Daranas, N., G. Rosello, J. Cabrefiga, I. Donati, J. Frances, E. Badosa, F. Spinelli, E. Montesinos and A. Bonaterra. 2019. Biological control of bacterial plant diseases with Lactobacillus plantarum strains selected for their broad-spectrum activity. Ann. Appl. Biol. 174: 92–105.
de Boer, M., P. Bom, F. Kindt, J. J. B. Keurentjes, I. van der Sluis, L. C. van Loon and P. A. H. M. Bakker. 2003. Control of Fusarium wilt of radish by combining Pseudomonas putida strains that have different disease-suppressive mechanisms. Biol. Control. 93: 626–632.
del Carmen, M., M. del Carmen Rocha-Granados and G. Glick. 2018. Microbiome engineering to improve biocontrol and plant growth promoting mechanisms. Microbiol. Res. 208: 25–31.
Di Giallonardo, F. and E. C. Holmes. 2015. Viral biocontrol: Grand experiments in disease emergence and evolution. Trends Microbiol. 23: 83–90. https://doi.org/10.1016/j.tim.2014.10.004
Diaz-Diaz, M., A. Bernal-Cabrera, A. Trapero, R. Medina-Marrero, S. Sifontes-Rodríguez, R. D. Cupull-Santana, M. García-Bernal and C. Agustí-Brisach. 2022. Characterization of actinobacterial strains as potential biocontrol agents against Macrophomina phaseolina and Rhizoctonia solani, the main soil-borne pathogens of Phaseolus vulgaris in Cuba. Plants. 11: 645.
Dobhal, A. and G. M. Hegde. 2021. Shelf life study of Pseudomonas fluorescens in liquid formulations. J. Farm Sci. 34(1): 84-86.
Downing, K. and J. A. Thomson. 2000. Introduction of the Serratia marcescens chiA 671 gene into an endophytic Pseudomonas fluorescens for the biocontrol of phytopathogenic fungi. Can. J. Microbiol. 46: 363–369.
Droby, S., E. Chalutz, R. Hofstein, C. L. Wilson, M. E. Wisniewski, B. Fridlender, L. Cohen, B. Weiss and A. Daus. 1993. Pilot testing of Pichia guilliermondii: a biocontrol agent of postharvest diseases of citrus fruit. Biol. Control. 3: 47–52.
Dunne, C., Y. Moenne-Loccoz, F. J. de Bruijin and F. O’Gara. 2000. Overproduction of an inducible extracellular serine protease improves biological control of Pythium ultimum by Stenotrophomonas maltophilia strain W81. Microbiology. 146: 2069–2078.
Dy, R. L., L. A. Rigano and P. C. Fineran. 2018. Phage-based biocontrol strategies and their application in agriculture and aquaculture. Biochem. Soc. Trans. 46: 1605–1613.
El-Tarabily, K. A. 2003. An endophytic chitinase-producing isolate of Actinoplanes missouriensis, with potential for biological control of root rot of lupine caused by Plectosporium tabacinum. Aust. J. Bot. 51: 257–266.
El-Tarabily, K. A., N. H. Soliman, A. H. Nassar, H. A. Al-Hassani, K. Sivasithamparam, F. Mc Kenna and G. E. Hardy. 2000. Biological control of Sclerotinia minor using a chitinolytic bacterium and actinomycetes. Plant Pathol. 49: 576–583.
El-Tarabily, K. A. 2006. Rhizosphere-competent isolates of streptomycete and non-streptomycete actinomycetes capable of producing cell-wall degrading enzymes to control Pythium aphanidermatum damping-off disease of cucumber. Can. J. Bot. 84: 211–222.
El-Tarabily, K. A., G. E. S. J. Hardy, K. Sivasithamparam, A. M. Hussein and I. D. KurtboE` ke. 1997. The potential for the biological control of cavity spot disease of carrots caused by Pythium coloratum by streptomycete and non-streptomycete actinomycetes in Western Australia. New Phytol. 137: 495–507.
Escande, A. R., F. S. Laich and M. V. Pedraza. 2002. Field testing of honeybee dispersed Trichoderma spp. to manage sunflower head rot (Sclerotinia sclerotiorum). Plant Pathol. 51: 346–351.
Esserti, S., A. Smaili, L. A. Rifai, T. Koussa, K. Makroum, M. Belfaiza, E. M. Kabil, L. Faize, L. Burgos and N. Alburquerque. 2017. Protective effect of three brown seaweed extracts against fungal and bacterial diseases of tomato. J. Appl. Phycol. 29: 1081–1093.
Fan, B., J. Blom, H. P. Klenk and R. Borriss. 2017. Bacillus amyloliquefaciens, Bacillus velezensis and Bacillus siamensis form an operational Group B. amyloliquefaciens within the B. subtilis species complex. Front Microbiol. 8: 22. https://doi.org/10.3389/fmicb.2017.00022
Farrar, K., D. Bryant and N. Cope-Selby. 2014. Understanding and engineering beneficial plant-microbe interactions: Plant growth promotion in energy crops. Plant Biotechnol. J. 12: 1193–1206.
Feklistova, I. N. and N. P. Maksimova. 2008. Obtaining Pseudomonas aurantiaca strains capable of overproduction of phenazine antibiotics. Microbiology. 77: 176–180.
Folimonova, S. Y. 2013. Developing an understanding of cross-protection by Citrus tristeza virus. Front Microbiol. 4: 76.
Folman, L. B., J. Postma and J. A. Van Veen. 2003. Inability to find consistent bacterial biocontrol agents of Pythium aphanidermatum in cucumber using screens based on ecophysiological traits. Microb. Ecol. 45: 72–87.
Fravel, D. R. 2005. Commercialization and implementation of biocontrol 1. Annu. Rev. Phytopathol. 43: 337–359.
García-Pedrajas, M. D., M. C. Cañizares, J. L. Sarmiento-Villamil, A. G. Jacquat and J. S. Dambolena. 2019. Mycoviruses in biological control: From basic research to field implementation. Phytopathology. 109: 1828–1839.
Ghorbanpour, M., M. Omidvari, P. Abbaszadeh-Dahaji, R. Omidvar and K. Kariman. 2018. Mechanisms underlying the protective effects of beneficial fungi against plant diseases. Biol. Control. 117: 147–157.
Hanson, L. E. 2000. Reduction of Verticillium wilt symptoms in cotton following seed treatment with Trichoderma virens. J. Cotton Sci. 4: 224–231.
He, D. C., M. H. He, D. M. Amalin, W. Liu, D. G. Alvindia and J. Zhan, 2021. Biological control of plant diseases: An evolutionary and eco-economic consideration. Pathogens. 10: 1311. https://doi.org/10.3390/pathogens10101311.
Hegde G. M. and L. H. Malligawad. 2020. Bioagents for the management of mildews in cucumber under protected cultivation. J. Mycol. Plant Pathol. 50(1): 1–10.
Hegde, G. M. and K. N. Vijaykumar. 2022. Formulation, application and commercialization of biopesticides in india. Asia Pacific Biofertilizers and Biopesticides Information Platform (APBB). https://apbb.fftc.org.tw/article/306. pp. 1-25.
Hegde, G. M. and K. N. Vijaykumar. 2023. Mechanisms of resistance of Trichoderma spp. against plant disease management. Asia Pacific Biofertilizers and Biopesticides Information Platform (APBB). https://apbb.fftc.org.tw/article/413 pp. 1-15.
Hegde, G. M. and S. Jahagirdar. 2020. Trichoderma: A potential millennium microbe for sustainable management of field and horticulture crop diseases: An overview. J. Farm Sci. 33(2): 159-172.
Hegde, G. M., A. Dobhal, K. N. Vijaykumar and S. Jahagirdar. 2023. Advances in formulations and efficacy of mycopesticides for plant disease management and sustainable yields. In: Fungal Resources for Sustainable Economy. Singh, I., V. R. Rajpal and S. S. Navi (eds) Springer, Singapore. https:/doi.org/10.1007/978-981-19-9103-5_14
Hegde, G. M., L. H. Malligawad, M. N. Sreenivasa and B. K. Chetri. 2022. Role of plant growth promoting microbes in the control of fungal foliar diseases of tomato under protected cultivation. Egypt. J. Biol. Pest Control. 32: 105. https://doi.org/10.1186/s41938-022-00606-7
Heungens, K. and J. Parke. 2000. Zoospore homing and infection events: effects of the biocontrol bacterium Burkholderia cepacia AMMDR1 on two oomycete pathogens of pea (Pisum sativum L.). Appl. Environ. Microbiol. 66: 5192–5200.
Hidayah, B. N. 2022. Biological control potential of Trichoderma species and bacterial antagonists against Sclerotinia sclerotiorum on canola in Western Australia. Int. J. Agric. Biol. 27: 215–227.
Hoeschle-Zeledon, I., P. Neuenschwander and L. Kumar. 2013. Regulatory challenges for biological control. SP-IPM Secretariat, International Institute of Tropical Agriculture (IITA), Ibadan, Nigeria. p. 43.
Hu, H., M. E. Wisniewski, A. Abdelfattah and X. Zheng. 2017. Biocontrol activity of a cold-adapted yeast from Tibet against grey mold in cherry tomato and its action mechanism. Extremophiles. 21: 789–803.
Hunziker, L., D. Beonisch, U. Groenhagen, A. Bailly, S. Schulz and L. Weisskopf. 2015. Pseudomonas strains naturally associated with potato plants produce volatiles with high potential for inhibition of Phytophthora infestans. Appl. Environ. Microbiol. 81: 821–830.
Ikeda, S., A. Shimizu, M. Shimizu, H. Takahashi and S. Takenaka. 2012. Biocontrol of black scurf on potato by seed tuber treatment with Pythium oligandrum. Biol. Control. 60: 297–304.
Iqbal, S. H., K. S. Qureshi and S. Ahmed. 1977. Influence of VA mycorrhiza on damping-off caused by Rhizoctonia solani in Brassica napus. Biologia. 23: 197–208.
Jadav, P. C., G. M. Hegde, S. Jahagirdar and V. Navi. 2022. Evaluation of microbial consortia against Sclerotium rolfsii Sacc. causing foot rot of wheat. J. Farm Sci. 35(2): 219-222.
Jahagirdar, S., G. M. Hegde, P. U. Krishnaraj and D. N. Kambrekar. 2021. Microbial consortia for plant disease management and sustainable productivity. In: Emerging Trends in Plant Pathology. Singh, K. P., S. Jahagirdar and B. K. Sarma (eds). Springer Nature Singapore Pvt. Ltd. https://doi.org/10.1007/978-981-15-6275-4
Jiang, Z., Y. Guo., S. Li, H. Qi and J. Guo. 2006. Evaluation of biocontrol efficiency of different Bacillus preparations and field application methods against Phytophthora blight of bell pepper. Biol. Control. 36: 216–223.
Jimenez, E., F. Dorta, C. Medina, A. Ramirez and I. Ramirez. 2011. Pena-Cortes, H. Anti-pathogenic activities of macro-algae extracts. Mar. Drugs. 9: 739–756.
Joshi, D., A. Kaushik, R. Kumar, A. Arya, G. Santoyo, V. K. Singh, N. Kashyap, M. K. Solanki, M. Kumari, N. Bhardwaj and A. Kumar. 2025. Improving plant performance through microbiome manipulation: The potential role of current bioengineering approaches. Bacteria. 4(1): 12.
Kamilova F, J. H. J. Leveau and B. J. J. Lugtenberg. 2007. Collimonas fungivorans, an unpredicted in vitro but efficient in vivo biocontrol agent for the suppression of tomato foot and root rot. Environ. Microbiol. 9: 597–1603.
Kasun, M., A. Dinushani, J. L. Alan, S. Phillips, D. Kannangara and P. Itthayakorn. 2020. Fungi vs fungi in biocontrol: An overview of fungal antagonists applied against fungal plant pathogens. Front. Cell. Infect. Microbiol. 10. https://doi.org/10.3389/fcimb.2020.604923
Kavino, M., S. Harish, N. Kumar, D. Saravanakumar and R. Samiyappan. 2008. Induction of systemic resistance in banana (Musa spp.) against Banana bunchy top virus (BBTV) by combining chitin with root-colonizing Pseudomonas fluorescens strain CHA0. Eur. J. Plant Pathol. 120: 353–362.
Khalifa, M. E. and R. M. MacDiarmid. 2021. A mechanically transmitted DNA mycovirus Is targeted by the defence machinery of its host, Botrytis cinerea. Viruses. 13: 1315.
Köhl, J., R. Kolnaar and W. J. Ravensberg. 2019. Mode of action of microbial biological control agents against plant diseases: Relevance beyond efficacy. Front. Plant Sci. 10: 845. doi: 10.3389/fpls.2019.00845.
Konstantinidou-Doltsinis, S., E. Markellou, N. Petsikos-Panayotarou, E. Siranidou, A. E. Kalamarakis, A. Schmitt, A. Ernst, B. Seddon, R. R. Belanger, A. J. Dik, D. Shtienberg. 2002. Biological control of fungal and bacterial plant pathogens. In: Combinations of biocontrol agents and Milsana(R) against powdery mildew and grey mould in cucumber in Greece and the Netherlands. Elad Y. and J. Kohl (eds). Vol 25. IOBC-WPRS Working Group, Kusadasi, pp. 171–174.
Koumoutsi, A., X. H. Chen, A. Henne, H. Liesegang, G. Hitzeroth, P. Franke, J. Vater and R. Borriss. 2004. Structural and functional characterization of gene clusters directing nonribosomal synthesis of bioactive cyclic lipopeptides in Bacillus amyloliquefaciens strain FZB42. J. Bacteriol. 186: 1084–1096.
Krishna, K. R. and D. J. Bagyaraj. 1983. Interaction between Glomus fasciculatus and Sclerotium rolfsii in peanut. Can. J. Bot. 67: 2349–2357.
Kumar, A., A. K. Chaturvedi, K. Yadav, K. P. Arunkumar, S. K. Malyan, P. Raja and A. N. Yadav. 2019. Fungal phytoremediation of heavy metal-contaminated resources: current scenario and future prospects. In: Recent Advancement. In: White Biotechnology Through Fungi. 437-461, Springer.
Kumar, V. and S. Chandel. 2016. Mycoviruses and their role in biological control of plant diseases. Int. J. Plant Sci. 11: 375–382.
Lahlali, R., S. Ezrari, N. Radouane, J. Kenfaoui, Q. Esmaeel, H. El Hamss, Z. Belabess and E. A. Barka. 2022. Biological control of plant pathogens: A global perspective. microorganisms, 10: 596. https://doi.org/10.3390/microorganisms10030596.
Lakkis, S., P. Trotel-Aziz, F. Rabenoelina, A. Schwarzenberg, E. Nguema-Ona, C. Clement and A. Aziz. 2019. Strengthening grapevine resistance by Pseudomonas fluorescens PTA-CT2 relies on distinct defense pathways in susceptible and partially resistant genotypes to downy mildew and grey mold diseases. Front. Plant Sci. 10: 1112.
Lecoq H. and J. M. Lemaire. 1991. Control of Zucchini yellow mosaic virus in squash by cross protection. Plant Dis. 75: 208–211.
Lherminier, J., N. Benhamou, J. Larrue, M. L. Milat, E. Boudon-Padieu, M. Nicole and J. P. Blein. 2003. Cytological characterization of elicitin-induced protection in tobacco plants infected by Phytophthora parasitica or Phytoplasma. Phytopathology. 93: 1308–1319.
Li, S., C. C. Jochum, F. Yu, K. Zaleta-Rivera, L. Du, S. D. Harris and G. Y. Yuen. 2008. An antibiotic complex from Lysobacter enzymogenes strain C3: antimicrobial activity and role in plant disease control. Phytopathology. 98: 695–701.
Lou, B. G., A. Y. Wang, C. Lin, T. Xu and X. D. Zheng. 2011. Enhancement of defense responses by oligandrin against Botrytis cinerea in tomatoes. Afr. J. Biotechnol. 10: 442–449.
Madsen, A. M. and E. de Neergaard. 1999. Interactions between the mycoparasite Pythium oligandrum and sclerotia of the plant pathogen Sclerotinia sclerotiorum. Eur. J. Plant Pathol. 105: 761–768.
Marco, J. L. D., M. C. Valadares-Inglis and C. R. Felix. 2003. Production of hydrolytic enzymes by Trichoderma isolates with antagonistic activity against Crinipellis perniciosa, the causal agent of witches’ broom of cocoa. Braz. J. Microbiol. 34: 33–38.
Marzano, M., A, Gallo and C. Altomare. 2013. Improvement of biocontrol efficacy of Trichoderma harzianum vs. Fusarium oxysporum f. sp. lycopersici through UV-induced tolerance to fusaric acid. Biol. Control. 67: 397–408.
Mateos, E., J. Olaizola, J. A. Pajares, V. Pando and J. J. Diez. 2017. Influence of Suillus luteus on Fusarium damping-off in pine seedlings. African J. Biotechnol. 16(6): 268-273.
Mishra, S., A. Singh, C. Keswani, A. Saxena, B. K. Sarma and H. B. Singh. 2015. Harnessing plant-microbe interactions for enhanced protection against phytopathogens. In plant microbes Symbiosis: Applied Facets. Springer, New Delhi, India, pp. 111–125.
Mishra, V., W. Ellouze and R. Howard. 2018. Utility of arbuscular mycorrhizal fungi for improved production and disease mitigation in organic and hydroponic greenhouse crops. J. Hortic. 5(3): 1000237. DOI:10.4172/2376-0354.1000237
Mouloud, G., H. Daoud, J. Bassem, I, Laribi Atef and B. Hani. 2013. New bacteriocin from Bacillus clausii strain GM17: Purification, characterization, and biological activity. Appl. Biochem. Biotechnol. 171: 2186–2200.
Murphy, J. F., G. W. Zehnder, D. J. Schuster, E. J. Sikora, J. E. Polston and J. W. Kloepper. 2000. Plant growth promoting rhizobacterial mediated protection in tomato against Tomato mottle virus. Plant Dis. 84: 779–784.
Naureen, Z., A. H. Price, F. Y. Hafeez and M. R. Roberts. 2009. Identification of rice blast disease suppressing bacterial strains from the rhizosphere of rice grown in Pakistan. Crop Prot. 28: 1052–1060.
Niranjan Raj, S., S. A. Deepak, P. Basavaraju, H. S. Shetty, M. S. Reddy and J. W. Kloepper. 2003. Comparative performance of formulations of plant growth promoting rhizobacteria in growth promotion and suppression of downy mildew in pearl millet. Crop Prot. 22: 579–588.
Orozco-Mosqueda, M. D. C., M. D. C. Rocha-Granados, B. R. Glick and G. Santoyo. 2018. Microbiome engineering to improve biocontrol and plant growth-promoting mechanisms. Microbiol. Res. 208: 25–31.
Osman, M. E. H., M. M. El-Sheekh, A. M. Metwally, I. Aea and M. Ismail. 2011. Antagonistic activity of some fungi and cyanobacteria species against Rhizoctonia solani. Int. J. Plant Pathol. 2: 101–114.
Palmieri, D., D. Vitullo, F. De Curtis and G. Lima, 2017, A microbial consortium in the rhizosphere as a new biocontrol approach against Fusarium decline of chickpea. Plant and Soil. 412: 425-439.
Palmieri, D., G. Barone, R. A. Cigliano, F. De Curtis, G. Lima, R. Castoria and G. Ianiri. 2021. Complete genome sequence of the biocontrol yeast Papiliotrema terrestris strain LS28. G3 Genes|Genomes|Genetics. 11: 332.
Pandit, M. A., J. Kumar, S. Gulati, N. Bhandari, P. Mehta, R. Katyal, C. D. Rawat, V. Mishra and J. Kaur. 2022. Major biological control strategies for plant pathogens. Pathogens. 11: 273. https://doi.org/10.3390/pathogens11020273
Parasuraman, P., S. Pattnaik and S. Busi. 2019. Phyllosphere microbiome: Functional importance in sustainable agriculture. In New and Future Developments in Microbial Biotechnology and Bioengineering. Singh, J. S. and D. P. Singh. Eds.; Elsevier: Amsterdam, The Netherlands, Chapter 10; pp. 135–148. ISBN 978-0-444-64191-5.
Peshin, R, R. S. Bandral, W. J. Zhang, et al. 2009. Integrated Pest Management: A Global Overview of History, Programs and Adoption. In: Integrated Pest Management: Innovation-Development Process (Vol. 1) (Peshin R, Dhawan AK, eds). 1-50, Springer, Netherlands.
Peshin, R. and W. J. Zhang. 2014. Integrated pest management and pesticide use. In: Integrated Pest Management: Pesticide Problems. Vol. 3. (eds, Pimentel, D. and R. Peshin). pp. 1-46. Springer, Netherlands.
Picard, K., M. Ponchet, J. P. Blein, P. Rey, Y. Tirilly and N. Benhamou. 2000. Oligandrin. A proteinaceous molecule produced by the mycoparasite Pythium oligandrum induces resistance to Phytophthora parasitica infection in tomato plants. Plant Physiol. 124: 379–395.
Pliego, C., S. de Weert, G. Lamers, A. de Vicente, G. V. Bloemberg, F. M. Cazorla and C. Ramos. 2008. Two similar enhanced root colonizing Pseudomonas strains differ largely in their colonization strategies of avocado roots and Rosellinia necatrix hyphae. Environ. Microbiol. 10: 3295–3304.
Prashant, P., Jambhulkar, P. Sharma and R. Yadav. 2016. Delivery systems for introduction of microbial inoculants in the field. In: Microbial Inoculants in Sustainable Agricultural Productivity. (eds. Singh D. P., et al.,). pp. 199–218.
Qu, Z., Y. Fu, Y. Lin, Z. Zhao, X. Zhang, J. Cheng, J. Xie, T. Chen, B. Li and D. Jiang. 2021. Transcriptional responses of Sclerotinia sclerotiorum to the infection by SsHADV-1. J. Fungi. 7: 493.
Ram, R. M., C. Keswani, K. Bisen, R. Tripathi, S. P. Singh and H. B. Singh. 2018. Biocontrol technology: Eco-friendly approaches for sustainable agriculture. In: Omics Technologies and Bio-Engineering. Academic Press. Cambridge, MA, USA, pp. 177–190.
Rekanovic, E., S. Milijasevic, B. Todorovic and I. Potocnik. 2007. Possibilities of biological and chemical control of Verticillium wilt in pepper. Phytoparasitica. 35: 436–441.
Risoli, S., L. Cotrozzi, S. Sarrocco, M. Nuzzaci, E. Pellegrini and A. Vitti. 2022. Trichoderma-induced resistance to Botrytis cinerea in Solanum species: A meta-analysis. Plants. 11: 180.
Roberti, R., S. Galletti, P. L. Burzi, H. Righini, S. Cetrullo and C. Perez. 2015. Induction of defence responses in zucchini (Cucurbita pepo) by Anabaena sp. water extract. Biol. Control. 82: 61–68.
Roca-Couso, R., J. D. Flores-Felix and R. Rivas. 2021. Mechanisms of action of microbial biocontrol agents against Botrytis cinerea. J. Fungi. 12: 1045.
https://doi.org/10.3390/jof7121045
Saikia, K., L. C. Bora, P. Bora and H. Hazarika. 2020. Management of bacterial blight in rice (Oryza sativa) through combined application of endophytes and rhizosphere antagonist. Indian J. Agric. Sci. 90(12): 2323–2327.
Saravanakumar, D., N. Lavanya, K. Muthumeena, T. Raguchander and R. Samiyappan. 2009. Fluorescent pseudomonad mixtures mediate disease resistance in rice plants against sheath rot (Sarocladium oryzae) disease. BioControl. 54: 273.
Savary, S., L. Willocquet, S. J. Pethybridge, P. Esker, N. McRoberts and A. Nelson. 2019. The global burden of pathogens and pests on major food crops. Nat. Ecol. Evol. 3: 430–439.
Saxena, A. K., H. Chakdar, M. Kumar, et al. 2021. ICAR technologies: Biopesticides for eco-friendly pest management. Indian Council of Agricultural Research, New Delhi.
Saxena, A. K., M. Kumar, H. Chakdar, N. Anuroopa and D. J. Bagyaraj. 2020. Bacillus species in soil as a natural resource for plant health and nutrition. J. Appl. Microbiol. 128: 1583–1594. https://doi.org/10.1111/jam.14506.
Silva, H. S. A., R. S. Romeiro, R. Carrer-Filho, J. L. A. Pereira, E. S. G. Mizubuti and A. Mounteer. 2004. Induction of systemic resistance by Bacillus cereus against tomato foliar diseases under field conditions. J. Phytopathol. 152: 371–375.
Simon, A. 1989. Biological control of take-all of wheat by Trichoderma koningii under controlled environmental conditions. Soil Biol. Biochem. 21: 323–326.
Singh, I. 2017. Arbuscular mycorrhiza mediated control of plant pathogens. In: Mycorrhiza—Nutrient Uptake, Biocontrol, Ecorestoration. (Varma, A., R. Prasad, and N. Tuteja. eds.) Springer, Cham, Switzerland.
Smith, G. E. 1957. Inhibition of Fusarium oxysporum f.sp. lycopersici by a species of Micromonospora isolated from tomato. Phytopathology. 47: 429–432.
Spadaro, D. and M. L. Gullino. 2004. State of the art and future prospects of the biological control of postharvest fruit diseases. Int. J. Food Microbiol. 91: 185–194.
Stenberg, J. A., I. Sundh, P. G. Becher, C. Bjorkman, M. Dubey, P. A. Egan, H. Friberg, J. F. Gil, D. F. Jensen, M. Jonsson, M. Karlsson, S. Khalil, V. Ninkovic, G. Rehermann, R. R. Vetukuri and M. Viketoft. 2021. When is it biological control? A framework of definitions, mechanisms, and classifications. J. Pest Sci. 94: 665–676.
https://doi.org/10.1007/s10340-021-01354-7
Sugiprihatini, D., S. Wiyono and S. Widodo. 2011. Selection of yeasts antagonists as biocontrol agent of mango fruit rot caused by Botryodiplodia theobromae. Microbiol Indones. 5: 154–159.
Sundh, I. and J. Eilenberg. 2020. Why has the authorization of microbial biological control agents been slower in the EU than in comparable jurisdictions. Pest Manag. Sci. 77: 2170–2178.
Swain, H., T. Adak, A. K. Mukherjee, P. K. Mukherjee, P. Bhattacharyya, S. Behera, T. B. Bagchi, R. P. Shasmita, A. Khandual, M. K. Bag, T. K. Dangar, S. Lenka and M. Jena. 2018. Novel Trichoderma strains isolated from tree barks as potential biocontrol agents and biofertilizers for direct seeded rice. Microbiol. Res. 214: 83-90. https://doi.org/10.1016/j.micres.2018.05.015.
Tahir, H. A. S., Q. Gu, H. Wu, Y. Niu, R. Huo and X. Gao. 2017. Bacillus volatiles adversely affect the physiology and ultra-structure of Ralstonia solanacearum and induce systemic resistance in tobacco against bacterial wilt. Sci. Rep. 7: 40481.
Takenaka, S. and S. Ishikawa. 2013. Biocontrol of sugar beet seedling and taproot diseases caused by Aphanomyces cochlioides by Pythium oligandrum treatments before transplanting. Jpn. Agric. Res. Quarter. 47: 75–83.
Takenaka, S. and H. Tamagake. 2009. Foliar spray of a cell wall protein fraction from the biocontrol agent Pythium oligandrum induces defence-related genes and increases resistance against Cercospora leaf spot in sugar beet. J. Gen. Plant Pathol. 75: 340–348.
Tennant, P. F., C. Gonsalves, K. S. Ling, M. Fitch, R. Manshardt, J. L. Slinghtom and D. Gonsalves. 1994. Differential protection against Papaya ring spot virus isolates in coat protein gene transgenic papaya and classically cross-protected papaya. Phytopathology. 84: 1359–1366.
Tilocca, B., A. Cao and Q. Migheli. 2020. Scent of a killer: Microbial volatilome and its role in the biological control of plant pathogens. Front. Microbiol. 11: 41.
Trappe, J. M. 1962. Fungus association of ectotrophic mycorrhizae. Bot. Rev. 28: 538-606.
Ugras, S., K. Sezen, H. Kati and Z. Demirbag. 2013. Purification and characterization of the bacteriocin Thuricin Bn1 produced by Bacillus thuringiensis subsp. kurstaki Bn1 isolated from a hazelnut pest. J. Microbiol. Biotechnol. 23: 167–176.
Vallance, J., G. Le Floch, F. Deniel, G. Barbier, C. A. Levesque and P. Rey. 2009. Influence of Pythium oligandrum biocontrol on fungal and oomycete population dynamics in the rhizosphere. Appl. Environ. Microbiol. 75: 4790–4800.
Veresoglou, S. D and M. C. Rillig. 2012. Suppression of fungal and nematode plant pathogens through arbuscular mycorrhizal fungi. Biol. Lett. 8: 214–217.
Vicedo, B., R. Penalver, M. J. Asins and M. M. Lopez. 1993. Biological control of Agrobacterium tumefaciens, colonization, and pAgK84 transfer with Agrobacterium radiobacter K84 and the Tra- mutant strain K1026. Appl. Environ. Microbiol. 59: 309–315.
Walterson, A. M. and J. Stavrinides. 2015. Pantoea: Insights into a highly versatile and diverse genus within the Enterobacteriaceae. FEMS Microbiol. Rev. 39: 968–984.
Walther, D. and D. Gindrat. 1988. Biological control of damping-off of sugar-beet and cotton with Chaetomium globosum or a fluorescent Pseudomonas sp. Can. J. Microbiol. 34: 631–637.
Weiss, A., G. Mögel and S. Kunz. 2006. Development of “Boni-Pro-tect”-a Yeast Preparation for Use in the Control of Post-harvest Diseases of Apples. In: Proceedings of the 12th International Conference on Cultivation Technique and Phytopathological Problems in Organic Fruit-Growing, Weinsberg, Germany, 31st January–2nd February 2006.
Wilson, M. J. and T. A. Jackson. 2013. Progress in the commercialisation of bionematicides. BioControl. 58: 715–722.
Wisniewski, M., C. Biles, S. Droby, R. McLaughlin, C. Wilson and E. Chalutz. 1991. Mode of action of the postharvest biocontrol yeast, Pichia guilliermondii. I. characterization of attachment to Botrytis cinerea. Physiol. Mol. Plant Pathol. 39: 245–258.
Wittmann, J., C. Brancato, K. W. Berendzen and B. Dreiseikelmann. 2016. Development of a tomato plant resistant to Clavibacter michiganensis using the endolysin gene of bacteriophage CMP1 as a transgene. Plant Pathol. 65: 496–502.
www.custommarketinsight.com
Xiao, K., L, Kinkel and D. A. Samac. 2002. Biological control of Phytophthora root rots on alfalfa and soybean with Streptomyces. Biol Control. 23: 285–295.
Xue, Y., Y. Zhang, K. Huang, X. Wang, M. Xing, Q. Xu and Y. Guo. 2023. A novel biocontrol agent Bacillus velezensis K01 for management of grey mold caused by Botrytis cinerea. AMB Express. 13: 91. doi: 10.1186/s13568-023-01596-x. PMID: 37642883; PMCID: PMC10465465.
Yao, H. J. and S. P. Tian. 2005. Effect of pre- and post-harvest application of salicylic acid or methyl jasmonate on inducing disease resistance of sweet cherry fruit in storage. Postharvest Biol. Technol. 35: 253–262.
You, M. P., K. Sivasithamparam, D. I. Kurtbeoke. 1996. Actinomycetes in organic mulch used in avocado plantations and their ability to suppress Phytophthora cinnamomi. Biol. Fertil. Soils. 22: 237–242.
Zehnder, G. W., J. F. Murphy, E. J. Sikora and J. W. Kloepper. 2001. Application of rhizobacteria for induced resistance. Eur. J. Plant Pathol. 107: 39–50.
Zehnder, G.W., C. Yao, J. F. Murphy, E. J. Sikora and J. W. Kloepper. 2000. Induction of resistance in tomato against cucumber mosaic cucumovirus by plant growth-promoting rhizobacteria. BioControl. 45: 127–137.
Zhang, J., D. V. Mavrodi, M. Yang, L. S. Thomashow, O. V. Mavrodi, J. Kelton and D. M. Weller. 2020. Pseudomonas synxantha 2–79 transformed with pyrrolnitrin biosynthesis genes has improved biocontrol activity against soilborne pathogens of wheat and canola. Phytopathol. 110: 1010–1017.
Zhang, S., A. L. Moyne, M. S. Reddy and J. W. Kloepper. 2002. The role of salicylic acid in induced systemic resistance elicited by plant growth-promoting rhizobacteria against blue mold of tobacco. Biol. Control. 25: 288–296.
Zhang, S., T. L. White, M. C. Martinez, J. A. McInroy, J. W. Kloepper and W. Klassen. 2010. Evaluation of plant growth-promoting rhizobacteria for control of Phytophthora blight on squash under greenhouse conditions. Biol. Control. 53: 129–135.
Zhang, W. J. 2018. Global pesticide use: Profile, trend, cost / benefit and more. Proc. Int. Acad. Ecol. Environ. Sci. 8(1): 1–27.
Zhang, W. J., F. B. Jiang and J. F. Ou. 2011. Global pesticide consumption and pollution: with China as a focus. Proc. Int. Acad. Ecol. Environ. Sci. 1(2): 125–144